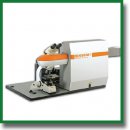
Learning from Nature: Bacterial Spores as a Target for Current Technologies in Medicine (Review)
The capability of some representatives of Clostridium spp. and Bacillus spp. genera to form spores in extreme external conditions long ago became a subject of medico-biological investigations. Bacterial spores represent dormant cellular forms of gram-positive bacteria possessing a high potential of stability and the capability to endure extreme conditions of their habitat. Owing to these properties, bacterial spores are recognized as the most stable systems on the planet, and spore-forming microorganisms became widely spread in various ecosystems.
Spore-forming bacteria have been attracted increased interest for years due to their epidemiological danger. Bacterial spores may be in the quiescent state for dozens or hundreds of years but after they appear in the favorable conditions of a human or animal organism, they turn into vegetative forms causing an infectious process. The greatest threat among the pathogenic spore-forming bacteria is posed by the causative agents of anthrax (B. anthracis), food toxicoinfection (B. cereus), pseudomembranous colitis (C.difficile), botulism (C. botulinum), gas gangrene (C. perfringens).
For the effective prevention of severe infectious diseases first of all it is necessary to study the molecular structure of bacterial spores and the biochemical mechanisms of sporulation and to develop innovative methods of detection and disinfection of dormant cells. There is another side of the problem: the necessity to investigate exo- and endospores from the standpoint of obtaining similar artificially synthesized models in order to use them in the latest medical technologies for the development of thermostable vaccines, delivery of biologically active substances to the tissues and intracellular structures. In recent years, bacterial spores have become an interesting object for the exploration from the point of view of a new paradigm of unicellular microbiology in order to study microbial heterogeneity by means of the modern analytical tools.
- Wang S., Shen A., Setlow P., Li Y.Q. Characterization of the dynamic germination of individual Clostridium difficile spores using Raman spectroscopy and differential interference contrast microscopy. J Bacteriol 2015; 197(14): 2361–2373, https://doi.org/10.1128/JB.00200-15.
- Rosales-Mendoza S., Angulo C., Meza B. Food-grade organisms as vaccine biofactories and oral delivery vehicles. Trends Biotechnol 2016; 34(2): 124–136, https://doi.org/10.1016/j.tibtech.2015.11.007.
- Barra-Carrasco J., Paredes-Sabja D. Clostridium difficile spores: a major threat to the hospital environment. Future Microbiol 2014; 9(4): 475–486, https://doi.org/10.2217/fmb.14.2.
- Abhyankar W., Pandey R., Ter Beek A., Brul S., De Koning L.J., De Koster C.G. Reinforcement of Bacillus subtilis spores by cross-linking of outer coat proteins during maturation. Food Microbiol 2015; 45 (Pt A): 54–62, https://doi.org/10.1016/j.fm.2014.03.007.
- Bressuire-Isoard C., Broussolle V., Carlin F. Sporulation environment influences spore properties in Bacillus: evidence and insights on underlying molecular and physiological mechanisms. FEMS Microbiol Rev 2018; 42(5): 614–626, https://doi.org/10.1093/femsre/fuy021.
- Nicholson W.L. Roles of Bacillus endospores in the environment. Cell Mol Life Sci 2002; 59(3): 410–416, https://doi.org/10.1007/s00018-002-8433-7.
- Paredes-Sabja D., Shen A., Sorg J.A. Clostridium difficile spore biology: sporulation, germination, and spore structural proteins. Trends Microbiol 2014; 22(7): 406–416, https://doi.org/10.1016/j.tim.2014.04.003.
- Rupnik M., Wilcox M.H., Gerding D.N. Clostridium difficile infection: new developments in epidemiology and pathogenesis. Nat Rev Microbiol 2009; 7(7): 526–536, https://doi.org/10.1038/nrmicro2164.
- Pizarro-Guajardo M., Chamorro-Veloso N., Vidal R.M., Paredes-Sabja D. New insights for vaccine development against Clostridium difficile infections. Anaerobe 2019; 58: 73–79, https://doi.org/10.1016/j.anaerobe.2019.04.009.
- Calderón-Romero P., Castro-Córdova P., Reyes-Ramírez R., Milano-Céspedes M., Guerrero-Araya E., Pizarro-Guajardo M., Olguín-Araneda V., Gil F., Paredes-Sabja D. Clostridium difficile exosporium cysteine-rich proteins are essential for the morphogenesis of the exosporium layer, spore resistance, and affect C. difficile pathogenesis. PLoS Pathog 2018; 14(8): e1007199, https://doi.org/10.1371/journal.ppat.1007199.
- McDonald L.C., Gerding D.N., Johnson S., Bakken J.S., Carroll K.C., Coffin S.E., Dubberke E.R., Garey K.W., Gould C.V., Kelly C., Loo V., Shaklee S.J., Sandora T.J., Wilcox M.H. Clinical practice guidelines for Clostridium difficile infection in adults and children: 2017 update by the Infectious Diseases Society of America (IDSA) and Society for Healthcare Epidemiology of America (SHEA). Clin Infect Dis 2018; 66(7): 987–994, https://doi.org/10.1093/cid/ciy149.
- Li A.G., Burggraf L.W., Xing Y. Nanomechanical characterization of Bacillus anthracis spores by atomic force microscopy. Appl Environ Microbiol 2016; 82(10): 2988–2999, https://doi.org/10.1128/AEM.00431-16.
- Fritze D. Taxonomy of the genus Bacillus and related genera: the aerobic endospore-forming bacteria. Phytopathology 2004; 94(11): 1245–1248, https://doi.org/10.1094/phyto.2004.94.11.1245.
- Stewart G.C. The exosporium layer of bacterial spores: a connection to the environment and the infected host. Microbiol Mol Biol Rev 2015; 79(4): 437–457, https://doi.org/10.1128/MMBR.00050-15.
- Tan I.S., Ramamurthi K.S. Spore formation in Bacillus subtilis. Environ Microbiol Rep 2014; 6(3): 212–225, https://doi.org/10.1111/1758-2229.12130.
- Steichen C., Kearney J.F., Turnbough C.L. Jr. Non-uniform assembly of the Bacillus anthracis exosporium and a bottle cap model for spore germination and outgrowth. Mol Microbiol 2007; 64(2): 359–367, https://doi.org/10.1111/j.1365-2958.2007.05658.x.
- Thompson B.M., Waller L.N., Fox K.F., Fox A., Stewart G.C. The BclB glycoprotein of Bacillus anthracis is involved in exosporium integrity. J Bacteriol 2007; 189(18): 6704–6713, https://doi.org/10.1128/jb.00762-07.
- Manetsberger J., Ghosh A., Hall E.A.H., Christie G. Orthologues of Bacillus subtilis spore crust proteins have a structural role in the Bacillus megaterium QM B1551 spore exosporium. Appl Environ Microbiol 2018; 84(20): e01734-18, https://doi.org/10.1128/aem.01734-18.
- Russell J.R., Cabeen M.T., Wiggins P.A., Paulsson J., Losick R. Noise in a phosphorelay drives stochastic entry into sporulation in Bacillus subtilis. EMBO J 2017; 36(19): 2856–2869, https://doi.org/10.15252/embj.201796988.
- Kailas L., Terry C., Abbott N., Taylor R., Mullin N., Tzokov S.B., Todd S.J., Wallace B.A., Hobbs J.K., Moir A., Bullough P.A. Surface architecture of endospores of the Bacillus cereus/anthracis/thuringiensis family at the subnanometer scale. Proc Natl Acad Sci U S A 2011; 108(38): 16014–16019, https://doi.org/10.1073/pnas.1109419108.
- Terry C., Shepherd A., Radford D.S., Moir A., Bullough P.A. YwdL in Bacillus cereus: its role in germination and exosporium structure. PLoS One 2011; 6: e23801, https://doi.org/10.1371/journal.pone.0023801.
- Cetinkaya Y., Yurt M.N.Z., Avni Oktem H., Yilmaz M.D. A monostyryl boradiazaindacene (BODIPY)-based lanthanide-free colorimetric and fluorogenic probe for sequential sensing of copper (II) ions and dipicolinic acid as a biomarker of bacterial endospores. J Hazard Mater 2019; 377: 299–304, https://doi.org/10.1016/j.jhazmat.2019.05.108.
- Errington J. Regulation of endospore formation in Bacillus subtilis. Nat Rev Microbiol 2003; 1(2): 117–126, https://doi.org/10.1038/nrmicro750.
- Giorno R., Bozue J., Cote C., Wenzel T., Moody K.S., Mallozzi M., Ryan M., Wang R., Zielke R., Maddock J.R., Friedlander A., Welkos S., Driks A. Morphogenesis of the Bacillus anthracis spore. J Bacteriol 2007; 189(3): 691–705, https://doi.org/10.1128/JB.00921-06.
- McKenney P.T., Driks A., Eichenberger P. The Bacillus subtilis endospore: assembly and functions of the multilayered coat. Nat Rev Micro 2013; 11(1): 33–44, https://doi.org/10.1038/nrmicro2921.
- Jiang S., Wan Q., Krajcikova D., Tang J., Tzokov S.B., Barak I., Bullough P.A. Diverse supramolecular structures formed by self-assembling proteins of the Bacillus subtilis spore coat. Mol Microbiol 2015; 97(2): 347–359, https://doi.org/10.1111/mmi.13030.
- Brunsing R.L., La Clair C., Tang S., Chiang C., Hancock L.E., Perego M., Hoch J.A. Characterization of sporulation histidine kinases of Bacillus anthracis. J Bacteriol 2005; 187(20): 6972–6981, https://doi.org/10.1128/jb.187.20.6972-6981.2005.
- Pratda V.G., Sanstad E.A., Wang R., Driks A. Morphogenesis of Bacillus spore surfaces. J Bacteriol 2003; 185(21): 6255–6261, https://doi.org/10.1128/jb.185.21.6255-6261.2003.
- Setlow P. Spores of Bacillus subtilis: their resistance to and killing by radiation, heat and chemicals. J Appl Microbiol 2006; 101(3): 514–525, https://doi.org/10.1111/j.1365-2672.2005.02736.x.
- Hong H.A., Hitri K., Hosseini S., Kotowicz N., Bryan D., Mawas F., Wilkinson A.J., van Broekhoven A., Kearsey J., Cutting S.M. Mucosal antibodies to the C terminus of toxin A prevent colonization of Clostridium difficile. Infect Immun 2017; 85(4): e01060-16, https://doi.org/10.1128/iai.01060-16.
- Widderich N., Rodrigues C.D.A., Commichau F.M., Fischer K.E., Ramirez-Guadiana F.H., Rudner D.Z., Bremer E. Salt-sensitivity of σH and Spo0A prevents sporulation of Bacillus subtilis at high osmolarity avoiding death during cellular differentiation. Mol Microbiol 2016; 100(1): 108–124, https://doi.org/10.1111/mmi.13304.
- Janganan T.K., Mullin N., Tzokov S.B., Stringer S., Fagan R.P., Hobbs J.K., Moir A., Bullough P.A. Characterization of the spore surface and exosporium proteins of Clostridium sporogenes; implications for Clostridium botulinum group I strains. Food Microbiol 2016; 59: 205–212, https://doi.org/10.1016/j.fm.2016.06.003.
- Bailey-Smith K., Todd S.J., Southworth T.W., Proctor J., Moir A. The ExsA protein of Bacillus cereus is required for assembly of coat and exosporium onto the spore surface. J Bacteriol 2005; 187(11): 3800–3806, https://doi.org/10.1128/jb.187.11.3800-3806.2005.
- Logan N.A. Bacillus and relatives in foodborne illness. J Appl Microbiol 2012; 112(3): 417–429, https://doi.org/10.1111/j.1365-2672.2011.05204.x.
- Hosoya S., Lu Z., Ozaki Y., Takeuchi M., Sato T. Cytological analysis of the mother cell death process during sporulation in Bacillus subtilis. J Bacteriol 2007; 189(6): 2561–2565, https://doi.org/10.1128/jb.01738-06.
- Setlow P. Spore resistance properties. Microbiol Spectr 2014; 2(5), https://doi.org/10.1128/microbiolspec.TBS-0003-2012.
- Batalha I.L., Ke P., Tejeda-Montes E., Uddin S., van der Walle C.F., Christie G. Dipicolinic acid as a novel spore-inspired excipient for antibody formulation. Int J Pharm 2017; 526(1–2): 332–338, https://doi.org/10.1016/j.ijpharm.2017.05.012.
- Scheldeman P., Herman L., Foster S., Heyndrickx M. Bacillus sporothermodurans and other highly heatresistant spore formers in milk. J Appl Microbiol 2006; 101(3): 542–555, https://doi.org/10.1111/j.1365-2672.2006.02964.x.
- Schubert W.W., Beaudet R.A. Determination of lethality rate constants and D-values for heat-resistant Bacillus spores ATCC 29669 exposed to dry heat from 125°C to 200°C. Astrobiology 2011; 11(3): 213–223, https://doi.org/10.1089/ast.2010.0502.
- Cheung M., Lee W.W., Cowcher D.P., Goodacre R., Bell S.E. SERS of meso-droplets supported on superhydrophobic wires allows exquisitely sensitive detection of dipicolinic acid, an anthrax biomarker, considerably below the infective dose. Chem Commun (Camb) 2016; 52(64): 9925–9928, https://doi.org/10.1039/c6cc03521c.
- Di Filippo P., Pomata D., Riccardi C., Buiarelli F., Uccelletti D., Zanni E. Muramic and dipicolinic acids in atmospheric particulate matter as biomarkers of bacteria and bacterial spores. Anal Bioanal Chem 2017; 409(6): 1657–1666, https://doi.org/10.1007/s00216-016-0111-y.
- Setlow P. I will survive: DNA protection in bacterial spores. Trends Microbiol 2007; 15(4): 172–180, https://doi.org/10.1016/j.tim.2007.02.004.
- Pestov D., Zhi M., Sariyanni Z.E., Kalugin N.G., Kolomenskii A.A., Murawski R., Paulus G.G., Sautenkov V.A., Schuessler H., Sokolov A.V., Welch G.R., Rostovtsev Y.V., Siebert T., Akimov D.A., Graefe S., Kiefer W., Scully M.O. Visible and UV coherent Raman spectroscopy of dipicolinic acid. Proc Natl Acad Sci U S A 2005; 102(42): 14976–14981, https://doi.org/10.1073/pnas.0506529102.
- Slieman T.A., Nicholson W.L. Role of dipicolinic acid in survival of Bacillus subtilis spores exposed to artificial and solar UV radiation. Appl Environ Microbiol 2001; 67(3): 1274–1279, https://doi.org/10.1128/aem.67.3.1274-1279.2001.
- Driks A. The dynamic spore. Proc Natl Acad Sci U S A 2003; 100(6): 3007–3009, https://doi.org/10.1073/pnas.0730807100.
- Wang C., Ehrhardt C.J., Yadavalli V.K. Single cell profiling of surface carbohydrates on Bacillus cereus. J R Soc Interface 2015; 12(103): 20141109, https://doi.org/10.1098/rsif.2014.1109.
- Bai X.R., Zeng Y., Zhou X.D., Wang X.H., Shen A.G., Hu J.M. Environmentally safe mercury(II) ions aided zero-background and ultrasensitive SERS detection of dipicolinic acid. Anal Chem 2017; 89(19): 10335–10342, https://doi.org/10.1021/acs.analchem.7b02172.
- Gao L., Zhao H., Li T., Huo P., Chen D., Liu B. Atomic force microscopy based tip-enhanced Raman spectroscopy in biology. Int J Mol Sci 2018; 19(4): E1193, https://doi.org/10.3390/ijms19041193.
- Wrobel T.P., Bhargava R. Infrared spectroscopic imaging advances as an analytical technology for biomedical sciences. Anal Chem 2018; 90(3): 1444–1463, https://doi.org/10.1021/acs.analchem.7b05330.
- Westphal A.J., Price P.B., Leighton T.J., Wheeler K.E. Kinetics of size changes of individual Bacillus thuringiensis spores in response to changes in relative humidity. Proc Natl Acad Sci U S A 2003; 100(6): 3461–3466, https://doi.org/10.1073/pnas.232710999.
- Higgins D., Dworkin J. Recent progress in Bacillus subtilis sporulation. FEMS Microbiol Rev 2012; 36(1): 131–148, https://doi.org/10.1111/j.1574-6976.2011.00310.x.
- Kong M., Na H., Ha N.C., Ryu S. LysPBC2, a novel endolysin harboring a Bacillus cereus spore binding domain. Appl Environ Microbiol 2019; 85(5): e02462-18, https://doi.org/10.1128/aem.02462-18.
- Lequette Y., Garénaux E., Combrouse T., Del Lima Dias T., Ronse A., Slomianny C., Trivelli X., Guerardel Y., Faille C. Domains of BclA, the major surface glycoprotein of the B. cereus exosporium: glycosylation patterns and role in spore surface properties. Biofouling 2011; 27(7): 751–761, https://doi.org/10.1080/08927014.2011.599842.
- Manetsberger J., Hall E.A.H., Christie G. Plasmid-encoded genes influence exosporium assembly and morphology in Bacillus megaterium QM B1551 spores. FEMS Microbiol Lett 2015; 362(18): fnv147, https://doi.org/10.1093/femsle/fnv147.
- Abhyankar W.R., Kamphorst K., Swarge B.N., van Veen H., van der Wel N.N., Brul S., de Koster C.G., de Koning L.J. The influence of sporulation conditions on the spore coat protein composition of Bacillus subtilis spores. Front Microbiol 2016; 7: 1636, https://doi.org/10.3389/fmicb.2016.01636.
- Checinska A., Paszczynski A., Burbank M. Bacillus and other spore-forming genera: variations in responses and mechanisms for survival. Annu Rev Food Sci Technol 2015; 6: 351–369, https://doi.org/10.1146/annurev-food-030713-092332.
- Bressuire-Isoard C., Bornard I., Henriques A.O., Carlin F., Broussolle V. Sporulation temperature reveals a requirement for CotE in the assembly of both the coat and exosporium layers of Bacillus cereus spores. Appl Environ Microbiol 2016; 82(1): 232–243, https://doi.org/10.1128/AEM.02626-15.
- Ireland J.A., Hanna P.C. Amino acid- and purine ribonucleoside-induced germination of Bacillus anthracis DeltaSterne endospores: gerS mediates responses to aromatic ring structures. J Bacteriol 2002; 184(5): 1296–1303, https://doi.org/10.1128/jb.184.5.1296-1303.2002.
- Cybulski R.J. Jr., Sanz P., McDaniel D., Darnell S., Bull R.L., O’Brien A.D. Recombinant Bacillus anthracis spore proteins enhance protection of mice primed with suboptimal amounts of protective antigen. Vaccine 2008; 26(38): 4927–4939, https://doi.org/10.1016/j.vaccine.2008.07.015.
- Giorno R., Mallozzi M., Bozue J., Moody K.S., Slack A., Qiu D., Wang R., Friedlander A., Welkos S., Driks A. Localization and assembly of proteins comprising the outer structures of the Bacillus anthracis spore. Microbiology 2009; 155: 1133–1145, https://doi.org/10.1099/mic.0.023333-0.
- Ramirez-Peralta A., Zhang P.F., Li Y.Q., Setlow P. Effects of sporulation conditions on the germination and germination protein levels of Bacillus subtilis spores. Appl Environ Microbiol 2012; 78(8): 2689–2697, https://doi.org/10.1128/aem.07908-11.
- Imamura D., Kuwana R., Takamatsu H., Watabe K. Proteins involved in formation of the outermost layer of Bacillus subtilis spores. J Bacteriol 2011; 193(16): 4075–4080, https://doi.org/10.1128/jb.05310-11.
- Terry C., Jiang S., Radford D.S., Wan Q., Tzokov S., Moir A., Bullough P.A. Molecular tiling on the surface of a bacterial spore — the exosporium of the Bacillus anthracis/cereus/thuringiensis group. Mol Microbiol 2017; 104(4): 539–552, https://doi.org/10.1111/mmi.13650.
- Barra-Carrasco J., Olguin-Araneda V., Plaza-Garrido A., Miranda-Cardenas C., Cofré-Araneda G., Pizarro-Guajardo M., Sarker M.R., Paredes-Sabja D. The Clostridium difficile exosporium cysteine (CdeC)-rich protein is required for exosporium morphogenesis and coat assembly. J Bacteriol 2013; 195(17): 3863–3875, https://doi.org/10.1128/jb.00369-13.
- Díaz-González F., Milano M., Olguin-Araneda V., Pizarro-Cerda J., Castro-Cordova P., Tzeng S.C., Maier C.S., Sarker M.R., Paredes-Sabja D. Protein composition of the outermost exosporium-like layer of Clostridium difficile 630 spores. J Proteomics 2015; 123: 1–13, https://doi.org/10.1016/j.jprot.2015.03.035.
- McPherson S.A., Li M., Kearney J.F., Turnbough C.L. Jr. ExsB, an unusually highly phosphorylated protein required for the stable attachment of the exosporium of Bacillus anthracis. Mol Microbiol 2010; 76(6): 1527–1538, https://doi.org/10.1111/j.1365-2958.2010.07182.x.
- Rodenburg C.M., McPherson S.A., Turnbough C.L. Jr., Dokland T. Cryo-EM analysis of the organization of BclA and BxpB in the Bacillus anthracis exosporium. J Struct Biol 2014; 186(1): 181–187, https://doi.org/10.1016/j.jsb.2014.02.018.
- Ehling-Schulz M., Lereclus D., Koehler T.M. The Bacillus cereus Group: Bacillus species with pathogenic potential. Microbiol Spectr 2019; 7(3), https://doi.org/10.1128/microbiolspec.gpp3-0032-2018.
- Pizarro-Guajardo M., Calderón-Romero P., Castro-Córdova P., Mora-Uribe P., Paredes-Sabja D. Ultrastructural variability of the exosporium layer of Clostridium difficile spores. Appl Environ Microbiol 2016; 82(7): 2202–2209, https://doi.org/10.1128/aem.03410-15.
- Pizarro-Guajardo M., Díaz-González F., Álvarez-Lobos M., Paredes-Sabja D. 622 characterization of chicken IgY specific to Clostridium difficile R20291 spores 623 and the effect of oral administration in mouse models of initiation and recurrent 624 disease. Front Cell Infect Microbiol 2017; 7: 365, https://doi.org/10.3389/fcimb.2017.00365.
- Pizarro-Guajardo M., Olguín-Araneda V., Barra-Carrasco J., Brito-Silva C., Sarker M.R., Paredes-Sabja D. Characterization of the collagen-like exosporium 634 protein, BclA1, of Clostridium difficile spores. Anaerobe 2014; 25: 18–30, https://doi.org/10.1016/j.anaerobe.2013.11.003.
- Pizarro-Guajardo M., Ravanal M.C., Paez M.D., Callegari E., Paredes-Sabja D. Identification of Clostridium difficile immunoreactive spore proteins of the epidemic strain R20291. Proteomics Clin Appl 2018; 12(5): e1700182, https://doi.org/10.1002/prca.201700182.
- Mora-Uribe P., Miranda-Cardenas C., Castro-Cordova P., Gil F., Calderón I., Fuentes J.A., Rodas P.I., Banawas S., Sarker M.R., Paredes-Sabja D. Characterization of the adherence of Clostridium difficile spores: the integrity of the outermost layer affects adherence properties of spores of the epidemic strain R20291 to components of the intestinal mucosa. Front Cell Infect Microbiol 2016; 6: 99, https://doi.org/10.3389/fcimb.2016.00099.
- Boydston J.A., Yue L., Kearney J.F., Turnbough C.L. The ExsY protein is required for complete formation of the exosporium of Bacillus anthracis. J Bacteriol 2006; 188: 7440–7448.
- Boydston J.A., Chen P., Steichen C.T., Turnbough C.L. Jr. Orientation within the exosporium and structural stability of the collagen-like glycoprotein BclA of Bacillus anthracis. J Bacteriol 2005; 187: 5310–5317, https://doi.org/10.1128/jb.00639-06.
- Brahmbhatt T.N., Janes B.K., Stibitz E.S., Darnell S.C., Sanz P., Rasmussen S.B., O’Brien A.D. Bacillus anthracis exosporium protein BclA affects spore germination, interaction with extracellular matrix proteins, and hydrophobicity. Infect Immun 2007; 75(11): 5233–5239, https://doi.org/10.1128/iai.00660-07.
- Brahmbhatt T.N., Darnell S.C., Carvalho H.M., Sanz P., Kang T.J., Bull R.L., Rasmussen S.B., Cross A.S., O’Brien A.D. Recombinant exosporium protein BclA of Bacillus anthracis is effective as a booster for mice primed with suboptimal amounts of protective antigen. Infect Immun 2007; 75(11): 5240–5247, https://doi.org/10.1128/iai.00884-07.
- Bozue J.A., Welkos S., Cote C.K. The Bacillus anthracis exosporium: what’s the big “hairy” deal? Microbiol Spectr 2015; 3(5), https://doi.org/10.1128/microbiolspec.tbs-0021-2015.
- Bozue J.А., Cote C.K., Moody K.L., Welkos S.L. Fully virulent Bacillus anthracis does not require the immunodominant protein BclA for pathogenesis. Infect Immun 2007; 75(1): 508–511, https://doi.org/10.1128/iai.01202-06.
- Ball D.A., Taylor R., Todd S.J., Redmond C., Couture-Tosi E., Sylvestre P. Structure of the exosporium and sublayers of spores of the Bacillus cereus family revealed by electron crystallography. Mol Microbiol 2008; 68(4): 947–958, https://doi.org/10.1111/j.1365-2958.2008.06206.x.
- Sylvestre P., Couture-Tosi E., Mock M. Polymorphism in the collagen-like region of the Bacillus anthracis BclA protein leads to variation in exosporium filament length. J Bacteriol 2003; 185: 1555–1563, https://doi.org/10.1128/jb.185.5.1555-1563.2003.
- Sylvestre P., Couture-Tosi E., Mock M. Contribution of ExsFA and ExsFB proteins to the localization of BclA on the spore surface and to the stability of the Bacillus anthracis exosporium. J Bacteriol 2005; 187(15): 5122–5128, https://doi.org/10.1128/jb.187.15.5122-5128.2005.
- Wang C., Stanciu C., Ehrhardt C.J., Yadavalli V.K. Morphological and mechanical imaging of Bacillus cereus spore formation at the nanoscale. J Microsc 2015; 258(1): 49–58, https://doi.org/10.1111/jmi.12214.
- Weaver J., Kang T.J., Raines K.W., Cao G.L., Hibbs S., Tsai P., Baillie L., Rosen G.M., Cross A.S. Protective role of Bacillus anthracis exosporium in macrophage-mediated killing by nitric oxide. Infect Immun 2007; 75(8): 3894–3901, https://doi.org/10.1128/iai.00283-07.
- Todd S.J., Moir A.J.G., Johnson M.J., Moir A. Genes of Bacillus cereus and Bacillus anthracis encoding proteins of the exosporium. J Bacteriol 2003; 185: 3373–3378, https://doi.org/10.1128/jb.185.11.3373-3378.2003.
- Wang Y., Jenkins S.A., Gu C., Shree A., Martinez-Moczygemba M., Herold J., Botto M., Wetsel R.A., Xu Y. Bacillus anthracis spore surface protein BclA mediates complement factor H binding to spores and promotes spore persistence. PLoS Pathog 2016; 12(6): e1005678, https://doi.org/10.1371/journal.ppat.1005678.
- Mehta A.S., Saile E., Zhong W., Buskas T., Carlson R., Kannenberg E., Reed Y., Quinn C.P., Boons G.J. Synthesis and antigenic analysis of the BclA glycoprotein oligosaccharide from the Bacillus anthracis exosporium. Chemistry 2006; 12(36): 9136–9149, https://doi.org/10.1002/chem.200601245.
- Johnson M.J., Todd S.J., Ball D.A., Shepherd A.M., Sylvestre P., Moir A. ExsY and CotY are required for the correct assembly of the exosporium and spore coat of Bacillus cereus. J Bacteriol 2006; 188(22): 7905–7913, https://doi.org/10.1128/jb.00997-06.
- Thompson B.M., Stewart G.C. Targeting of the BclA and BclB proteins to the Bacillus anthracis spore surface. Mol Microbiol 2008; 70(2): 421–434, https://doi.org/10.1111/j.1365-2958.2008.06420.x.
- Thompson B.M., Hsieh H.Y., Spreng K.A., Stewart G.C. The co-dependence of BxpB/ExsFA and BclA for proper incorporation into the exosporium of Bacillus anthracis. Mol Microbiol 2011; 79(3): 799–813, https://doi.org/10.1111/j.1365-2958.2010.07488.x.
- Thompson B.M., Hoelscher B.C., Driks A., Stewart G.C. Assembly of the BclB glycoprotein into the exosporium and evidence for its role in the formation of the exosporium ‘cap’ structure in Bacillus anthracis. Mol Microbiol 2012; 86(5): 1073–1084, https://doi.org/10.1111/mmi.12042.
- Steichen C., Kearney J.F., Turnbough C.L. Jr. Characterization of the exosporium basal layer protein BxpB of Bacillus anthracis. J Bacteriol 2005; 187(17): 5868–5876, https://doi.org/10.1128/jb.187.17.5868-5876.2005.
- Severson K.M., Mallozzi M., Bozue J., Welkos S.L., Cote C.K., Knight K.L., Driks A. Roles of the Bacillus anthracis spore protein ExsK in exosporium maturation and germination. J Bacteriol 2009; 191(24): 7587–7596, https://doi.org/10.1128/jb.01110-09.
- Boone T.J., Mallozzi M., Nelson A., Thompson B., Khemmani M., Lehmann D., Dunkle A., Hoeprich P., Rasley A., Stewart G., Driks A. Coordinated assembly of the Bacillus anthracis coat and exosporium during bacterial spore outer layer formation. MBio 2018; 9(6): e01166-18, https://doi.org/10.1128/mbio.01166-18.
- Lanzilli M., Donadio G., Addevico R., Saggese A., Cangiano G., Baccigalupi L., Christie G., Ricca E., Isticato R. The exosporium of Bacillus megaterium QM B1551 is permeable to the red fluorescence protein of the coral Discosoma sp. Front Microbiol 2016; 7: 1752, https://doi.org/10.3389/fmicb.2016.01752.
- McKenney P.T., Driks A., Eskandarian H.A., Grabowski P., Guberman J., Wang K.H., Gitai Z., Eichenberger P. A distance-weighted interaction map reveals a previously uncharacterized layer of the Bacillus subtilis spore coat. Curr Biol 2010; 20(10): 934–938, https://doi.org/10.1016/j.cub.2010.03.060.
- Shuster B., Khemmani M., Abe K., Huang X., Nakaya Y., Maryn N., Buttar S., Gonzalez A.N., Driks A., Sato T., Eichenberger P. Contributions of crust proteins to spore surface properties in Bacillus subtilis. Mol Microbiol 2019; 111(3): 825–843, https://doi.org/10.1111/mmi.14194.
- Yan X., Gai Y., Liang L., Liu G., Tan H. A gene encoding alanine racemase is involved in spore germination in Bacillus thuringiensis. Arch Microbiol 2007; 187(5): 371–378, https://doi.org/10.1007/s00203-006-0201-x.
- Chesnokova O.N., McPherson S.A., Steichen C.T., Turnbough C.L. Jr. The spore-specific alanine racemase of Bacillus anthracis and its role in suppressing germination during spore development. J Bacteriol 2009; 191(4): 1303–1310, https://doi.org/10.1128/jb.01098-08.
- Driks A. The Bacillus anthracis spore. Mol Aspects Med 2009; 30(6): 368–373, https://doi.org/10.1016/j.mam.2009.08.001.
- Horii T., Notake S., Tamai K., Yanagisawa H. Bacillus cereus from blood cultures: virulence genes, antimicrobial susceptibility and risk factors for blood stream infection. FEMS Immunol Med Microbiol 2011; 63(2): 202–209, https://doi.org/10.1111/j.1574-695x.2011.00842.x.
- Gauthier Y.P., Tournier J.N., Paucod J.C., Corre J.P., Mock M., Goossens P.L., Vidal D.R. Efficacy of a vaccine based on protective antigen and killed spores against experimental inhalational anthrax. Infect Immun 2009; 77(3): 1197–1207, https://doi.org/10.1128/iai.01217-08.
- Ghose C., Eugenis I., Edwards A.N., Sun X., McBride S.M., Ho D.D. Immunogenicity and protective efficacy of Clostridium difficile spore proteins. Anaerobe 2016; 37: 85–95, https://doi.org/10.1016/j.anaerobe.2015.12.001.
- Vedantam G., Kochanowsky J., Lindsey J., Mallozzi M., Roxas J.L., Adamson C., Anwar F., Clark A., Claus-Walker R., Mansoor A., McQuade R., Monasky R.C., Ramamurthy S., Roxas B., Viswanathan V.K. An engineered synthetic biologic protects against Clostridium difficile infection. Front Microbiol 2018; 9: 2080, https://doi.org/10.3389/fmicb.2018.02080.
- Driks A., Eichenberger P. The spore coat. Microbiol Spectr 2016; 4(2): 1–22, https://doi.org/10.1128/microbiolspec.tbs-0023-2016.
- Sorg J.A., Sonenshein A.L. Bile salts and glycine as cogerminants for Clostridium difficile spores. J Bacteriol 2008; 190(7): 2505–2512, https://doi.org/10.1128/jb.01765-07.
- Dubberke E.R., Olsen M.A. Burden of Clostridium difficile on the healthcare system. Clin Infect Dis 2012; 55(Suppl 2): S88–S92, https://doi.org/10.1093/cid/cis335.
- Driks A. Surface appendages of bacterial spores. Mol Microbiol 2007; 63(3) 623–625, https://doi.org/10.1111/j.1365-2958.2006.05564.x.
- Edwards A.N., McBride S.M. Initiation of sporulation in Clostridium difficile: a twist on the classic model. FEMS Microbiol Lett 2014; 358(2): 110–118, https://doi.org/10.1111/1574-6968.12499.
- Oliva C.R., Swiecki M.K., Griguer C.E., Lisanby M.W., Bullard D.C., Turnbough C.L. Jr., Kearney J.F. The integrin Mac-1 (CR3) mediates internalization and directs Bacillus anthracis spores into professional phagocytes. Proc Natl Acad Sci U S A 2008; 105(4): 1261–1266, https://doi.org/10.1073/pnas.0709321105.
- Tournier J.N., Ulrich R.G., Quesnel-Hellmann A., Mohamadzadeh M., Stiles B.G. Anthrax, toxins and vaccines: a 125-year journey targeting Bacillus anthracis. Expert Rev Anti-Infect Ther 2009; 7(2): 219–236, https://doi.org/10.1586/14787210.7.2.219.
- Perego M., Hoch J.A. Commingling regulatory systems following acquisition of virulence plasmids by Bacillus anthracis. Trends Microbiol 2008; 16(5): 215–221, https://doi.org/10.1016/j.tim.2008.01.010.
- Basoli F., Giannitelli S.M., Gori M., Mozetic P., Bonfanti A., Trombetta M., Rainer A. Biomechanical characterization at the cell scale: present and prospects. Front Physiol 2018; 9: 1449, https://doi.org/10.3389/fphys.2018.01449.
- Yoo L., Reed J., Shin A., Demer J.L. Atomic force microscopy determination of young’s modulus of bovine extra-ocular tendon fiber bundles. J Biomech 2014; 47(8): 1899–1903, https://doi.org/10.1016/j.jbiomech.2014.02.011.
- Alsteens D., Müller D.J., Dufrêne Y.F. Multiparametric atomic force microscopy imaging of biomolecular and cellular systems. Acc Chem Res 2017; 50(4): 924–931, https://doi.org/10.1021/acs.accounts.6b00638.
- Bolshakova A.V., Kiselyova O.I., Yaminsky I.V. Microbial surfaces investigated using atomic force microscopy. Biotechnol Prog 2004; 20(6): 1615–1622, https://doi.org/10.1021/bp049742c.
- Prats-Mateu B., Gierlinger N. Tip in-light on: advantages, challenges, and applications of combining AFM and Raman microscopy on biological samples. Microsc Res Tech 2017; 80(1): 30–40, https://doi.org/10.1002/jemt.22744.
- Gavara N. A beginner’s guide to atomic force microscopy probing for cell mechanics. Microsc Res Tech 2017; 80(1): 75–84, https://doi.org/10.1002/jemt.22776.
- Scheuring S., Dufrêne Y.F. Atomic force microscopy: probing the spatial organization, interactions and elasticity of microbial cell envelopes at molecular resolution. Mol Microbiol 2010; 75(6): 1327–1336, https://doi.org/10.1111/j.1365-2958.2010.07064.x.
- Müller D.J., Dufrêne Y.F. Atomic force microscopy as a multifunctional molecular toolbox in nanobiotechnology. Nat Nanotechnol 2008; 3(5): 261−269, https://doi.org/10.1038/nnano.2008.100.
- Xiao J., Dufrêne Y.F. Optical and force nanoscopy in microbiology. Nat Microbiol 2016; 1(11): 16186, https://doi.org/10.1038/nmicrobiol.2016.186.
- Gordon V.D., Wang L. Bacterial mechanosensing: the force will be with you, always. J Cell Sci 2019; 132(7): jcs227694, https://doi.org/10.1242/jcs.227694.
- Morisaku T., Kido Y., Asai K., Yui H. Mechanical properties of the coat protein layer and cortex in single Bacillus subtilis spores studied with an atomic force microscope and laser-induced surface deformation microscope. Anal Sci 2019; 35(1): 45–48, https://doi.org/10.2116/analsci.18sdp02.
- Iturri J., Toca-Herrera J.L. Characterization of cell scaffolds by atomic force microscopy. Polymers (Basel) 2017; 9(8): E383, https://doi.org/10.3390/polym9080383.
- Zolock R.A., Li G., Bleckmann C., Burggraf L.W., Fuller D.C. Atomic force microscopy of Bacillus spore surface morphology. Micron 2006; 37(4): 363–369, https://doi.org/10.1016/j.micron.2005.11.006.
- Dufrêne Y.F. Atomic force microscopy in microbiology: new structural and functional insights into the microbial cell surface. MBio 2014; 5(4): e01363-14, https://doi.org/10.1128/mbio.01363-14.
- Chiorcea-Paquim A.M., Eritja R., Oliveira-Brett A.M. Electrochemical and AFM characterization of G-Quadruplex electrochemical biosensors and applications. J Nucleic Acids 2018; 2018: 5307106, https://doi.org/10.1155/2018/5307106.
- Benitez R., Toca-Herrera J.L. Looking at cell mechanics with atomic force microscopy: experiment and theory. Microsc Res Tech 2014; 77(11): 947–958, https://doi.org/10.1002/jemt.22419.
- Fisher N., Hanna P. Characterization of Bacillus anthracis germinant receptors in vitro. J Bacteriol 2005; 187(23): 8055–8062, https://doi.org/10.1128/jb.187.23.8055-8062.2005.
- Xing Y., Li A., Felker D.L., Burggraf L.W. Nanoscale structural and mechanical analysis of Bacillus anthracis spores inactivated with rapid dry heating. Appl Environ Microbiol 2014; 80(5): 1739–1749, https://doi.org/10.1128/aem.03483-13.
- Cote C.K., Bozue J., Moody K.L., DiMezzo T.L., Chapman C.E., Welkos S.L. Analysis of a novel spore antigen in Bacillus anthracis that contributes to spore opsonization. Microbiology 2008; 154(Pt 2): 619–632, https://doi.org/10.1099/mic.0.2007/008292-0.
- Fox A., Stewart G.C., Wallera L.N., Fox K.F., Harley W.M., Price R.L. Carbohydrates and glycoproteins of Bacillus anthracis and related bacilli: targets for biodetection. J Microbiol Methods 2003; 54(2): 143–152, https://doi.org/10.1016/s0167-7012(03)00095-2.
- Chan J.W., Esposito A.P., Talley C.E., Hollars C.W., Lane S.M., Huser T. Reagentless identification of single bacterial spores in aqueous solution by confocal laser tweezers Raman spectroscopy. Anal Chem 2004; 76(3): 599–603, https://doi.org/10.1021/ac0350155.
- Evanoff D.D. Jr., Heckel J., Caldwell T.P., Christensen K.A., Chumanov G. Monitoring DPA release from a single germinating Bacillus subtilis endospore via surface-enhanced Raman scattering microscopy. J Am Chem Soc 2006; 128(39): 12618–12619, https://doi.org/10.1021/ja0642717.
- Daniels J.K., Caldwell T.P., Christensen K.A., Chumanov G. Monitoring the kinetics of Bacillus subtilis endospore germination via surface-enhanced Raman scattering spectroscopy. Anal Chem 2006; 78(5): 1724–1729, https://doi.org/10.1021/ac052009v.
- Huang S.S., Chen D., Pelczar P.L., Vepachedu V.R., Setlow P., Li Y.Q. Levels of Ca2+-dipicolinic acid in individual Bacillus spores determined using microfluidic Raman tweezers. J Bacteriol 2007; 189(13): 4681–4687, https://doi.org/10.1128/jb.00282-07.
- Wang S., Doona C.J., Setlow P., Li Y.Q. Use of Raman spectroscopy and phase-contrast microscopy to characterize cold atmospheric plasma inactivation of individual bacterial spores. Appl Environ Microbiol 2016; 82(19): 5775–5784, https://doi.org/10.1128/aem.01669-16.
- Boitor R., Sinjab F., Strohbuecker S., Sottile V., Notingher I. Towards quantitative molecular mapping of cells by Raman microscopy: using AFM for decoupling molecular concentration and cell topography. Faraday Discuss 2016; 187: 199–212, https://doi.org/10.1039/c5fd00172b.
- Jung H., Kim D.B., Gweon B., Moon S.Y., Choe W. Enhanced inactivation of bacterial spores by atmospheric pressure plasma with catalyst TiO2. Appl Catal B-Environ 2010; 93(3–4): 212–216, https://doi.org/10.1016/j.apcatb.2009.09.031.
- Shintani H. Inactivation of bacterial spore, endotoxin, lipid A, normal prion and abnormal prion by exposures to several sorts of gases plasma. Biocontrol Sci 2016; 21(1): 1–12, https://doi.org/10.4265/bio.21.1.
- López M., Calvo T., Prieto M., Múgica-Vidal R., Muro-Fraguas I., Alba-Elías F., Alvarez-Ordóñez A. A review on non-thermal atmospheric plasma for food preservation: mode of action, determinants of effectiveness, and applications. Front Microbiol 2019; 10: 622, https://doi.org/10.3389/fmicb.2019.00622.
- Hertwig C., Steins V., Reineke K., Rademacher A., Klocke M., Rauh C., Schlüter O. Impact of surface structure and feed gas composition on Bacillus subtilis endospore inactivation during direct plasma treatment. Front Microbiol 2015; 6: 774, https://doi.org/10.3389/fmicb.2015.00774.
- Schlüter O., Ehlbeck J., Hertel C., Habermeyer M., Roth A., Engel K.H., Holzhauser T., Knorr D., Eisenbrand G. Opinion on the use of plasma processes for treatment of foods. Mol Nutr Food Res 2013; 57(5): 920–927, https://doi.org/10.1002/mnfr.201300039.
- van Bokhorst-van de Veen H., Xie H., Esveld E., Abee T., Mastwijk H., Nierop Groot M. Inactivation of chemical and heat-resistant spores of Bacillus and Geobacillus by nitrogen cold atmospheric plasma evokes distinct changes in morphology and integrity of spores. Food Microbiol 2015; 45(Pt A): 26–33, https://doi.org/10.1016/j.fm.2014.03.018.
- Reineke K., Langer K., Hertwig C., Ehlbeck J., Schlüter O. The impact of different process gas compositions on the inactivation effect of an atmospheric pressure plasma jet on Bacillus spores. Innov Food Sci Emerg Technol 2015; 30: 112–118, https://doi.org/10.1016/j.ifset.2015.03.019.
- Klämpfl T.G., Isbary G., Shimizu T., Li Y.F., Zimmermann J.L., Stolz W., Schlegel J., Morfill G.E., Schmidt H.U. Cold atmospheric air plasma sterilization against spores and other microorganisms of clinical interest. Appl Environ Microbiol 2012; 78(15): 5077–5082, https://doi.org/10.1128/aem.00583-12.
- Takamatsu T., Uehara K., Sasaki Y., Hidekazu M., Matsumura Y., Iwasawa A., Ito N., Kohno M., Azuma T., Okino A. Microbial inactivation in the liquid phase induced by multigas plasma jet. PLoS One 2015; 10(7): e0132381, https://doi.org/10.1371/journal.pone.0132381.
- Tseng S., Abramzon N., Jackson J.O., Lin W.J. Gas discharge plasmas are effective in inactivating Bacillus and Clostridium spores. Appl Microbiol Biotechnol 2012; 93(6): 2563–2570, https://doi.org/10.1007/s00253-011-3661-0.
- Ercan U.K., Smith J., Ji H.F., Brooks A.D., Joshi S.G. Chemical changes in nonthermal plasma-treated N-acetylcysteine (NAC) solution and their contribution to bacterial inactivation. Sci Rep 2016; 6: 20365, https://doi.org/10.1038/srep20365.
- Ricca E., Cutting S.M. Emerging applications of bacterial spores in nanobiotechnology. J Nanobiotechnology 2003; 1(1): 6, https://doi.org/10.1186/1477-3155-1-6.
- Minton N.P. Clostridia in cancer therapy. Nat Rev Microbiol 2003; 1(3): 237–242, https://doi.org/10.1038/nrmicro777.
- Bengtsson-Palme J., Kristiansson E., Larsson D.G.J. Environmental factors influencing the development and spread of antibiotic resistance. FEMS Microbiol Rev 2018; 42(1), https://doi.org/10.1093/femsre/fux053.
- Uyen N.Q., Hong H.A., Cutting S.M. Enhanced immunisation and expression strategies using bacterial spores as heat-stable vaccine delivery vehicles. Vaccine 2007; 25(2): 356–365, https://doi.org/10.1016/j.vaccine.2006.07.025.
- Roberts N.J., Zhang L., Janku F., Collins A., Bai R.Y., Staedtke V., Rusk A.W., Tung D., Miller M., Roix J., Khanna K.V., Murthy R., Benjamin R.S., Helgason T., Szvalb A.D., Bird J.E., Roy-Chowdhuri S., Zhang H.H., Qiao Y., Karim B., McDaniel J., Elpiner A., Sahora A., Lachowicz J., Phillips B., Turner A., Klein M.K., Post G., Diaz L.A. Jr., Riggins G.J., Papadopoulos N., Kinzler K.W., Vogelstein B., Bettegowda C., Huso D.L., Varterasian M., Saha S., Zhou S. Intratumoral injection of Clostridium novyi-NT spores induces antitumor responses. Sci Transl Med 2014; 6(249): 249ra111, https://doi.org/10.1126/scitranslmed.3008982.
- Heap J.T., Theys J., Ehsaan M., Kubiak A.M., Dubois L., Paesmans K., Van Mellaert L., Knox R., Kuehne S.A., Lambin P., Minton N.P. Spores of Clostridium engineered for clinical efficacy and safety cause regression and cure of tumors in vivo. Oncotarget 2014; 5(7): 1761–1769, https://doi.org/10.18632/oncotarget.1761.
- Sibley L., Reljic R., Radford D.S., Huang J.M., Hong H.A., Cranenburgh R.M., Cutting S.M. Recombinant Bacillus subtilis spores expressing MPT64 evaluated as a vaccine against tuberculosis in the murine model. FEMS Microbiol Lett 2014; 358(2): 170–179, https://doi.org/10.1111/1574-6968.12525.
- Das K., Thomas T., Garnica O., Dhandayuthapani S. Recombinant Bacillus subtilis spores for the delivery of Mycobacterium tuberculosis Ag85B-CFP10 secretory antigens. Tuberculosis (Edinb) 2016; 101S: S18–S27, https://doi.org/10.1016/j.tube.2016.09.016.
- Duc le H., Hong H.A., Fairweather N., Ricca E., Cutting S.M. Bacterial spores as vaccine vehicles. Infect Immun 2003; 71(5): 2810–2818, https://doi.org/10.1128/iai.71.5.2810-2818.2003.
- Farjadian F., Moghoofei M., Mirkiani S., Ghasemi A., Rabiee N., Hadifar S., Beyzavi A., Karimi M., Hamblin M.R. Bacterial components as naturally inspired nano-carriers for drug/gene delivery and immunization: set the bugs to work? Biotechnol Adv 2018; 36(4): 968–985, https://doi.org/10.1016/j.biotechadv.2018.02.016.
- Paul C., Filippidou S., Jamil I., Kooli W., House G.L., Estoppey A., Hayoz M., Junier T., Palmieri F., Wunderlin T., Lehmann A., Bindschedler S., Vennemann T., Chain P.S.G., Junier P. Bacterial spores, from ecology to biotechnology. Adv Appl Microbiol 2019; 106: 79–111, https://doi.org/10.1016/bs.aambs.2018.10.002.
- Shoemaker W.R., Lennon J.T. Evolution with a seed bank: the population genetic consequences of microbial dormancy. Evol Appl 2018; 11(1): 60–75, https://doi.org/10.1111/eva.12557.
- Tetz G., Tetz V. Introducing the sporobiota and sporobiome. Gut Pathog 2017; 9: 38, https://doi.org/10.1186/s13099-017-0187-8.