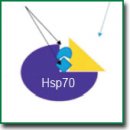
Новые тенденции использования терапевтической гипотермии как метода нейропротекции при повреждениях головного мозга (обзор)
В обзоре проанализированы современные клинические исследования использования терапевтической гипотермии в качестве нейропротективного метода лечения повреждений головного мозга. Этот метод демонстрирует хорошие результаты при острых повреждениях головного мозга, а также у пациентов в хронических критических состояниях. Показан интерес исследователей к изучению превентивных возможностей терапевтической гипотермии при вторичных повреждениях нейронов. Описано участие в механизмах нейропротекции головного мозга новых молекул, несущих положительные эффекты для тканей и клеток ЦНС, — белков и гормонов холодового стресса. Рассматриваются перспективы использования регулируемой целевой температуры (targeted temperature management) в лечении повреждений головного мозга.
- Jones W.H.S., Withington E.T. (translators). Hippocrates. De Vetere Medicina. Loeb Classical Library; 460–375 BC.
- Dzięcioł M., Kacprzak M., Goleniewska B., Zielińska M. Osborn wave in patients with ST-elevation myocardial infarction undergoing mild hypothermia after cardiac arrest. Acta Cardiol 2014; 69(5): 532–540, https://doi.org/10.2143/AC.69.5.3044880.
- Dell’Anna A.M., Taccone F.S., Halenarova K., Citerio G. Sedation after cardiac arrest and during therapeutic hypothermia. Minerva Anestesiol 2014; 80(8): 954–962.
- Phelps C. Principles of treatment. In: Traumatic injuries of the brain and its membranes. New York: D. Appleton and Co.; 1897.
- Karnatovskaia L.V., Wartenberg K.E., Freeman W.D. Therapeutic hypothermia for neuroprotection: history, mechanisms, risks and clinical applications. Neurohospitalist 2014; 4(3): 153–163, https://doi.org/10.1177/1941874413519802.
- Jackson T.C., Kochanek P.M. A new vision for therapeutic hypothermia in the era of targeted temperature management: a speculative synthesis. Ther Hypothermia Temp Manag 2019; 9(1): 13–47, https://doi.org/10.1089/ther.2019.0001.
- Martinello K., Hart A.R., Yap S., Mitra S., Robertson N.J. Management and investigation of neonatal encephalopathy: 2017 update. Arch Dis Child Fetal Neonatal Ed 2017; 102(4): F346–F358, https://doi.org/10.1136/archdischild-2015-309639.
- Nielsen N., Wetterslev J., Cronberg T., Erlinge D., Gasche Y., Hassager C., Horn J., Hovdenes J., Kjaergaard J., Kuiper M., Pellis T., Stammet P., Wanscher M., Wise M.P., Åneman A., Al-Subaie N., Boesgaard S., Bro-Jeppesen J., Brunetti I., Bugge J.F., Hingston C.D., Juffermans N.P., Koopmans M., Køber L., Langørgen J., Lilja G., Møller J.E., Rundgren M., Rylander C., Smid O., Werer C., Winkel P., Friberg H.; TTM Trial Investigators. Targeted temperature management at 33°C versus 36°C after cardiac arrest. N Engl J Med 2013; 369(23): 2197–2206, https://doi.org/10.1056/NEJMoa1310519.
- Kuroda Y., Kawakita K. Targeted temperature management for postcardiac arrest syndrome. J Neurocrit Care 2020; 13(1): 1–18, https://doi.org/10.18700/jnc.200001.
- Callaway C.W., Donnino M.W., Fink E.L., Geocadin R.G., Golan E., Kern K.B., Leary M., Meurer W.J., Peberdy M.A., Thompson T.M., Zimmerman J.L. Part 8: post-cardiac arrest care: American Heart Association guidelines update for cardiopulmonary resuscitation and emergency cardiovascular care. Circulation 2015: 132(18 Suppl 2): S465–S482, https://doi.org/10.1161/CIR.0000000000000262.
- Hanel R.A., Spetzler R.F. Surgical treatment of complex intracranial aneurysms. Neurosurgery 2008; 62(6 Suppl 3): 1289–1297, https://doi.org/10.1227/01.neu.0000333794.13844.d9.
- Tian D.H., Wan B., Bannon P.G., Misfeld M., LeMaire S.A., Kazui T., Kouchoukos N.T., Elefteriades J.A., Bavaria J., Coselli J.S., Greipp R.B., Mohr F.W., Oo A., Svensson L.G., Hughes G.C., Yan T.D. A meta-analysis of deep hypothermic circulatory arrest versus moderate hypothermic circulatory arrest with selective antegrade cerebral perfusion. Ann Cardiothorac Surg 2013; 2(2): 148–158, https://doi.org/10.3978/j.issn.2225-319X.2013.03.13.
- Шевелев О.А., Гречко А.В., Петрова М.В. Терапевтическая гипотермия. М: РУДН; 2019.
- Phadtare S., Alsina J., Inouye M. Cold-shock response and cold-shock proteins. Curr Opin Microbiol 1999; 2(2): 175–180, https://doi.org/10.1016/S1369-5274(99)80031-9.
- Jinka T.R., Tøien O., Drew K.L. Season primes the brain in an arctic hibernator to facilitate entrance into torpor mediated by adenosine A1 receptors. J Neurosci 2011; 31: 10752–10758, https://doi.org/10.1523/JNEUROSCI.1240-11.2011.
- Leonov Y., Sterz F., Safar P., Radovsky A., Oku K., Tisherman S., Stezoski S.W. Mild cerebral hypothermia during and after cardiac arrest improves neurologic outcome in dogs. J Cereb Blood Flow Metab 1990; 10(1): 57–70, https://doi.org/10.1038/jcbfm.1990.8.
- Ridenour T.R., Warner D.S., Todd M.M., McAllister A.C. Mild hypothermia reduces infarct size resulting from temporary but not permanent focal ischemia in rats. Stroke 1992; 23(5): 733–738, https://doi.org/10.1161/01.str.23.5.733.
- Hakim S.M., Ammar M.A., Reyad M.S. Effect of therapeutic hypothermia on survival and neurological outcome in adults suffering cardiac arrest: a systematic review and meta-analysis. Minerva Anestesiol 2018; 84(6): 720–730, https://doi.org/10.23736/S0375-9393.18.12164-X.
- Yum S.K., Seo Y.M., Kwun Y., Moon C.J., Youn Y.A., Sung I.K. Therapeutic hypothermia in infants with hypoxic-ischemic encephalopathy and reversible persistent pulmonary hypertension: short-term hospital outcomes. Matern Fetal Neonatal Med 2018; 31(23): 3108–3114, https://doi.org/10.1080/14767058.2017.1365123.
- Leng L. Hypothermia therapy after traumatic brain injury: a systematic review and meta-analysis. Turk Neurosurg 2017, https://doi.org/10.5137/1019-5149.JTN.19696-16.2.
- Bernal A., Arranz L. Nestin-expressing progenitor cells: function, identity and therapeutic implications. Cell Mol Life Sci 2018; 75(12): 2177–2195, https://doi.org/10.1007/s00018-018-2794-z.
- Mignone J.L., Kukekov V., Chiang A.S., Steindler D., Enikolopov G. Neural stem and progenitor cells in nestin-GFP transgenic mice. Comp Neurol 2004; 469(3): 311–324, https://doi.org/10.1002/cne.10964.
- Tubulin beta 3 class III. URL: https://www.ncbi.nlm.nih.gov/gene/10381.
- Kaufmann A.M., Firlik A.D., Fukui M.B., Wechsler L.R., Jungries C.A., Yonas H. Ischemic core and penumbra in human stroke. Stroke 1999; 30(1): 93–99, https://doi.org/10.1161/01.str.30.1.93.
- Coles J.P., Fryer T.D., Smielewski P., Chatfield D.A., Steiner L.A. Incidence and mechanisms of cerebral ischemia in early clinical head injury. J Cereb Blood Flow Metab 2004; 24(2): 202–211, https://doi.org/10.1097/01.WCB.0000103022.98348.24.
- Iordanova B., Li L., Clark R.S.B., Manole M.D. Alterations in cerebral blood flow after resuscitation from cardiac arrest. Front Pediatr 2017; 5: 174, https://doi.org/10.3389/fped.2017.00174.
- Edgar A., Bering J. Effect of body temperature change on cerebral oxygen consumption of the intact monkey. Am J Physiol 1961; 200: 417–419, https://doi.org/10.1152/ajplegacy.1961.200.3.417.
- Steen P.A., Newberg L., Milde J.H., Michenfelder J.D. Hypothermia and barbiturates: individual and combined effects on canine cerebral oxygen consumption. Anesthesiology 1983; 58(6): 527–532.
- Metz C., Holzschuh M., Bein T., Woertgen C., Frey A., Frey I., Taeger K., Brawanski A. Moderate hypothermia in patients with severe head injury: cerebral and extracerebral effects. J Neurosurg 1996; 85(4): 533–541, https://doi.org/10.3171/jns.1996.85.4.0533.
- Kohen R., Nyska A. Oxidation of biological systems: oxidative stress phenomena, antioxidants, redox reactions, and methods for their quantification. Toxicol Pathol 2002; 30(6): 620–650, https://doi.org/10.1080/01926230290166724.
- Lei B., Adachi N., Arai T. The effect of hypothermia on H2O2 production during ischemia and reperfusion: a microdialysis study in the gerbil hippocampus. Neurosci Lett 1997; 222(2): 91–94, https://doi.org/10.1016/s0304-3940(97)13349-3.
- Globus M.Y., Alonso O., Dietrich W.D., Busto R., Ginsberg M.D. Glutamate release and free radical production following brain injury: effects of posttraumatic hypothermia. J Neurochem 1995; 65(4): 1704–1711, https://doi.org/10.1046/j.1471-4159.1995.65041704.x.
- Chatzipanteli K., Wada K., Busto R., Dietrich W.D. Effects of moderate hypothermia on constitutive and inducible nitric oxide synthase activities after traumatic brain injury in the rat. J Neurochem 1999; 72(5): 2047–2052, https://doi.org/10.1046/j.1471-4159.1999.0722047.x.
- Bayir H., Adelson P.D., Wisniewski S.R., Shore P., Lai Y., Brown D., Janesko-Feldman K.L., Kagan V.E., Kochanek P.M. Therapeutic hypothermia preserves antioxidant defenses after severe traumatic brain injury in infants and children. Crit Care Med 2009; 37(2): 689–695, https://doi.org/10.1097/CCM.0b013e318194abf2.
- Hackenhaar F.S., Medeiros T.M., Heemann F.M., Behling C.S., Putti J.S., Mahl C.D., Verona C., da Silva A.C.A., Guerra M.C., Gonçalves C.A.S., Oliveira V.M., Riveiro D.F.M., Vieira S.R.R., Benfato M.S. Therapeutic hypothermia reduces oxidative damage and alters antioxidant defenses after cardiac arrest. Oxid Med Cell Longev 2017; 2017: 8704352, https://doi.org/10.1155/2017/8704352.
- Chamoun R., Suki D., Gopinath S.P., Goodman J.C., Robertson C. Role of extracellular glutamate measured by cerebral microdialysis in severe traumatic brain injury. J Neurosurg 2010; 113(3): 564–570, https://doi.org/10.3171/2009.12.JNS09689.
- Schober A., Warenits A.M., Testori C., Weihs W., Hosmann A., Högler S., Sterz F., Janata A., Scherer T., Magnet I.A., Ettl F., Laggner A.N., Herkner H., Zeitlinger M. Microdialysis assessment of cerebral perfusion during cardiac arrest, extracorporeal life support and cardiopulmonary resuscitation in rats — a pilot trial. PloS One 2016; 11(5): e0155303, https://doi.org/10.1371/journal.pone.0155303.
- Gouix E., Léveillé F., Nicole O., Melon C., Aissouni L., Buisson A. Reverse glial glutamate uptake triggers neuronal cell death through extrasynaptic NMDA receptor activation. Mol Cell Neurosci 2009; 40(4): 463–473, https://doi.org/10.1016/j.mcn.2009.01.002.
- Hardingham G.E., Fukunaga Y., Bading H. Extrasynaptic NMDARs oppose synaptic NMDARs by triggering CREB shut-off and cell death pathways. Nat Neurosci 2002; 5(5): 405–414, https://doi.org/10.1038/nn835.
- Suehiro E., Fujisawa H., Ito H., Ishikawa T., Maekawa T. Brain temperature modifies glutamate neurotoxicity in vivo. J Neurotrauma 1999; 16(4): 285–297, https://doi.org/10.1089/neu.1999.16.285.
- Mitani A., Kataoka K. Critical levels of extracellular glutamate mediating gerbil hippocampal delayed neuronal death during hypothermia: brain microdialysis study. Neuroscience 1991; 42(3): 661–670, https://doi.org/10.1016/0306-4522(91)90035-m.
- Campos F., Pérez-Mato M., Agulla J., Blanco M., Barral D., Almeida A., Brea D., Waeber C., Castillo J., Ramos-Cabrer P. Glutamate excitoxicity is the key molecular mechanism which is influenced by body temperature during the acute phase of brain stroke. PloS One 2012; 7(8): e44191, https://doi.org/10.1371/journal.pone.0044191.
- Shuaib A., Kanthan R., Goplen G., Griebel R., el-Azzouni H., Miyashita H., Liu L., Hogan T. In-vivo microdialysis study of extracellular glutamate response to temperature variance in subarachnoid hemorrhage. Acta Neurochir Suppl 1996; 67: 53–58, https://doi.org/10.1007/978-3-7091-6894-3_12.
- Schubert G.A., Poli S., Mendelowitsch A., Schilling L., Thomé C. Hypothermia reduces early hypoperfusion and metabolic alterations during the acute phase of massive subarachnoid hemorrhage: a laser-Doppler-flowmetry and microdialysis study in rats. Neurotrauma 2008; 25(5): 539–548, https://doi.org/10.1089/neu.2007.0500.
- Irazuzta J.E., Zingarelli B., Milam K., Kiefaber M., Kamdar T. Hypothermia attenuates inflammation in bacterial meningitis. Pediatr Res 1999; 45: 41a, https://doi.org/10.1203/00006450-199904020-00250.
- Frugier T., Morganti-Kossmann M.C., O’Reilly D., McLean C.A. In situ detection of inflammatory mediators in post mortem human brain tissue after traumatic injury. Neurotrauma 2010; 27(3): 497–507, https://doi.org/10.1089/neu.2009.1120.
- Ansari M.A. Temporal profile of M1 and M2 responses in the hippocampus following early 24 h of neurotrauma. J Neurol Sci 2015; 357(1–2): 41–49, https://doi.org/10.1016/j.jns.2015.06.062.
- Garcia J.H., Liu K.F., Yoshida Y., Lian J., Chen S., del Zoppo G.J. Influx of leukocytes and platelets in an evolving brain infarct (Wistar rat). Am J Pathol 1994; 144(1): 188–199.
- Price C.J., Menon D.K., Peters A.M., Ballinger J.R., Barber R.W. Cerebral neutrophil recruitment, histology, and outcome in acute ischemic stroke: an imaging-based study. Stroke 2004; 35(7): 1659–1664, https://doi.org/10.1161/01.STR.0000130592.71028.92.
- Harting M.T., Jimenez F., Adams S.D., Mercer D.W., Cox C.S. Jr. Acute, regional inflammatory response after traumatic brain injury: implications for cellular therapy. Surgery 2008; 144(5): 803–813, https://doi.org/10.1016/j.surg.2008.05.017.
- Boddaert J., Bielen K., Jongers B., Manocha E., Yperzeele L., Cras P., Pirici D., Kumar-Singh S. CD8 signaling in microglia/ macrophage M1 polarization in a rat model of cerebral ischemia. PloS One 2018; 13(1): e0186937, https://doi.org/10.1371/journal.pone.0186937.
- Loane D.J., Kumar A. Microglia in the TBI brain: the good, the bad, and the dysregulated. Exp Neurol 2016; 275(Pt 3): 316–327, https://doi.org/10.1016/j.expneurol.2015.08.018.
- Truettner J.S., Bramlett H.M., Dietrich W.D. Posttraumatic therapeutic hypothermia alters microglial and macrophage polarization toward a beneficial phenotype. J Cereb Blood Flow Metab 2017; 37(8): 2952–2962, https://doi.org/10.1177/0271678X16680003.
- Liu L.Q., Liu X.R., Zhao J.Y., Yan F., Wang R.L., Wen S.H., Wang L., Luo Y.M., Ji X.M. Brain-selective mild hypothermia promotes long-term white matter integrity after ischemic stroke in mice. CNS Neurosci Ther 2018; 24(12): 1275–1285, https://doi.org/10.1111/cns.13061.
- Sweeney M.D., Zhao Z., Montagne A., Nelson A.R., Zlokovic B.V. Blood-brain barrier: from physiology to disease and back. Physiol Rev 2019; 99(1): 21–78, https://doi.org/10.1152/physrev.00050.2017.
- Wennberg R.P., Hance A.J. Experimental bilirubin encephalopathy: importance of total bilirubin, protein binding, and blood-brain barrier. Pediatr Res 1986; 20(8): 789–792, https://doi.org/10.1203/00006450-198608000-00018.
- Kristensson K. Microbes’ roadmap to neurons. Nat Rev Neurosci 2011; 12(6): 345–357, https://doi.org/10.1038/nrn3029.
- Rifkind J.M., Mohanty J.G., Nagababu E. The pathophysiology of extracellular hemoglobin associated with enhanced oxidative reactions. Front Physiol 2014; 5: 500, https://doi.org/10.3389/fphys.2014.00500.
- Smith S.L., Hall E.D. Mild pre- and posttraumatic hypothermia attenuates blood-brain barrier damage following controlled cortical impact injury in the rat. Neurotrauma 1996; 13(1): 1–9, https://doi.org/10.1089/neu.1996.13.1.
- Lotocki G., Vaccari J.P.D., Perez E.R., Sanchez-Molano J., Furones-Alonso O., Bramlett H.M., Dietrich W.D. Alterations in blood-brain barrier permeability to large and small molecules and leukocyte accumulation after traumatic brain injury: effects of post-traumatic hypothermia. Neurotrauma 2009; 26(7): 1123–1134, https://doi.org/10.1089/neu.2008.0802.
- Tang X.N., Liu L., Koike M.A., Yenari M.A. Mild hypothermia reduces tissue plasminogen activator-related hemorrhage and blood brain barrier disruption after experimental stroke. Ther Hypothermia Temp Manag 2013; 3(2): 74–83, https://doi.org/10.1089/ther.2013.0010.
- Liu Y.C., Lee Y.D., Wang H.L., Liao K.H., Chen K.B., Poon K.S., Pan Y.L., Lai T.W. Anesthesia-induced hypothermia attenuates early-phase blood-brain barrier disruption but not infarct volume following cerebral ischemia. PloS One 2017; 12(1): e0170682, https://doi.org/10.1371/journal.pone.0170682.
- Irazuzta J.E., Pretzlaff R., Rowin M., Milam K., Zemlan F.P., Zingarelli B. Hypothermia as an adjunctive treatment for severe bacterial meningitis. Brain Res 2000; 881(1): 88–97, https://doi.org/10.1016/s0006-8993(00)02894-8.
- Song F., Guo C., Geng Y., Wu X., Fan W. Therapeutic time window and regulation of autophagy by mild hypothermia after intracerebral hemorrhage in rats. Brain Res 2018; 1690: 12–22, https://doi.org/10.1016/j.brainres.2018.04.005.
- Lee J.E., Yoon Y.J., Moseley M.E., Yenari M.A. Reduction in levels of matrix metalloproteinases and increased expression of tissue inhibitor of metalloproteinase-2 in response to mild hypothermia therapy in experimental stroke. J Neurosurg 2005; 103(2): 289–297, https://doi.org/10.3171/jns.2005.103.2.0289.
- Li J., Li C., Yuan W., Wu J., Zhao Y. Mild hypothermia alleviates brain oedema and blood-brain barrier disruption by attenuating tight junction and adherens junction breakdown in a swine model of cardiopulmonary resuscitation. PloS One 2017; 12(3): e0174596, https://doi.org/10.1371/journal.pone.0174596.
- Choi J.S., Park J., Suk K., Moon C., Park Y.K., Han H.S. Mild hypothermia attenuates intercellular adhesion molecule-1 induction via activation of extracellular signal-regulated kinase-1/2 in a focal cerebral ischemia model. Stroke Res Treat 2011; 2011: 846716, https://doi.org/10.4061/2011/846716.
- Edwards A.D., Yue X., Squier M.V., Thoresen M., Cady E.B., Penrice J., Cooper C.E., Wyatt J.S., Reynolds E.O., Mehmet H. Specific inhibition of apoptosis after cerebral hypoxiaischaemia by moderate post-insult hypothermia. Biochem Biophys Res Commun 1995; 217(3): 1193–1199, https://doi.org/10.1006/bbrc.1995.2895.
- Eroğlu O., Deniz T., Kisa Ü., Atasoy P., Aydinuraz K. Effect of hypothermia on apoptosis in traumatic brain injury and hemorrhagic shock model. Injury 2017; 48(12): 2675–2682, https://doi.org/10.1016/j.injury.2017.09.032.
- Liebetrau M., Burggraf D., Martens H.K., Pichler M., Hamann G.F. Delayed moderate hypothermia reduces calpain activity and breakdown of its substrate in experimental focal cerebral ischemia in rats. Neurosci Lett 2004; 357(1): 17–20, https://doi.org/10.1016/j.neulet.2003.12.031.
- Lu J., Qian H.Y., Liu L.J., Zhou B.C., Xiao Y., Mao J.N., An G.Y., Rui M.Z., Wang T., Zhu C.L. Mild hypothermia alleviates excessive autophagy and mitophagy in a rat model of asphyxial cardiac arrest. Neurol Sci 2014; 35(11): 1691–1699, https://doi.org/10.1007/s10072-014-1813-6.
- Liu T., Zhao D.X., Cui H., Chen L., Bao Y.H., Wang Y., Jiang J.Y. Therapeutic hypothermia attenuates tissue damage and cytokine expression after traumatic brain injury by inhibiting necroptosis in the rat. Sci Rep 2016; 6: 24547, https://doi.org/10.1038/srep24547.
- Tomura S., de Rivero Vaccari J.P., Keane R.W., Bramlett H.M., Dietrich W.D. Effects of therapeutic hypothermia on inflammasome signaling after traumatic brain injury. Cereb Blood Flow Metab 2012; 32(10): 1939–1947, https://doi.org/10.1038/jcbfm.2012.99.
- Zhou M., Wang P., Yang Z., Wu H., Huan Z. Spontaneous hypothermia ameliorated inflammation and neurologic deficit in rat cardiac arrest models following resuscitation. Mol Med Rep 2018; 17(2): 2127–2136, https://doi.org/10.3892/mmr.2017.8113.
- Ellis R.J. The molecular chaperone concept. Semin Cell Biol 1990; 1(1): 1–9.
- Hartl F.U. Molecular chaperones in cellular protein folding. Nature 1996; 381(6583): 571–580, https://doi.org/10.1038/381571a0.
- Giffard R.G., Xu L., Zhao H., Carrico W., Ouyang Y., Qiao Y., Sapolsky R., Steinberg G., Hu B., Yenari M.A. Chaperones, protein aggregation, and brain protection from hypoxic/ischemic injury. J Exp Biol 2004; 207(Pt 18): 3213–3220, https://doi.org/10.1242/jeb.01034.
- Beere H.M. “The stress of dying”: the role of heat shock proteins in the regulation of apoptosis. J Cell Sci 2004; 117(Pt 13): 2641–2651, https://doi.org/10.1242/jcs.01284.
- Muchowski P.J., Wacker J.L. Modulation of neurodegeneration by molecular chaperones. Nat Rev Neurosci 2005; 6(1): 11–22, https://doi.org/10.1038/nrn1587.
- Voellmy R. On mechanisms that control heat shock transcription factor activity in metazoan cells. Cell Stress Chaperones 2004; 9(2): 122–133, https://doi.org/10.1379/csc-14r.1.
- Fernandez-Funez P., Rincon-Limas D.E. Launching Hsp70 neuroprotection: two drugs better than one. Cell Cycle 2014; 13(11): 1657–1658, https://doi.org/10.4161/cc.29148.
- Yenari M.A. Heat shock proteins and neuroprotection. Adv Exp Med Biol 2002; 513: 281–299, https://doi.org/10.1007/978-1-4615-0123-7_10.
- Calderwood S.K., Mambula S.S., Gray P.J. Jr., Theriault J.R. Extracellular heat shock proteins in cell signaling. FEBS Lett 2007; 581(19): 3689–3694, https://doi.org/10.1016/j.febslet.2007.04.044.
- Sharma H.S. Hyperthermia induced brain oedema: current status and future perspectives. Indian J Med Res 2006; 123(5): 629–652.
- Vishwakarma S.K., Paspala S.A.B., Tiwari S.K., Khan A. ATP-binding cassette (ABC) transporters as emerging targets in modulation of neural stem cell behavior in neurodegenerative diseases and cell therapy benefits. JCMR 2014; 6(1): 44–49, https://doi.org/10.22067/jcmr.v6i1.30375.
- Mrozek S., Vardon F., Geeraerts T. Brain temperature: physiology and pathophysiology after brain injury. Anesthesiol Res Pract 2012; 2012: 989487, https://doi.org/10.1155/2012/989487.
- Ornitz D.M., Itoh N. The fibroblast growth factor signaling pathway. Wiley Interdiscip Rev Dev Biol 2015; 4(3): 215–266, https://doi.org/10.1002/wdev.176.
- Thompson L.D., Pantoliano M.W., Springer B.A. Energetic characterization of the basic fibroblast growth factor-heparin interaction: identification of the heparin binding domain. Biochemistry 1994; 33(13): 3831–3840, https://doi.org/10.1021/bi00179a006.
- Itoh N., Ornitz D.M. Functional evolutionary history of the mouse Fgf gene family. Dev Dyn 2008; 237(1): 18–27, https://doi.org/10.1002/dvdy.21388.
- Chen G.Z., Liu Y., Goetz R., Fu L.L., Jayaraman S., Hu M.C., Moe O.W., Liang G., Li X., Mohammadi M. α-Klotho is a nonenzymatic molecular scaffold for FGF23 hormone signalling. Nature 2018; 553(7689): 461–466, https://doi.org/10.1038/nature25451.
- Lee S., Choi J., Mohanty J., Sousa L.P., Tome F., Pardon E., Steyaert J., Lemmon M.A., Lax I., Schlessinger J. Structures of β-klotho reveal a ‘zip code’-like mechanism for endocrine FGF signalling. Nature 2018; 553(7689): 501–505, https://doi.org/10.1038/nature25010.
- Kurosu H., Choi M., Ogawa Y., Dickson A.S., Goetz R., Eliseenkova A.V., Mohammadi M., Rosenblatt K.P., Kliewer S.A., Kuro-o M. Tissue-specific expression of βKlotho and fibroblast growth factor (FGF) receptor isoforms determines metabolic activity of FGF19 and FGF21. Biol Chem 2007; 282(37): 26687–26695, https://doi.org/10.1074/jbc.M704165200.
- Adams A.C., Cheng C.C., Coskun T., Kharitonenkov A. FGF21 requires βklotho to act in vivo. PLoS One 2012; 7(11): e49977, https://doi.org/10.1371/journal.pone.0049977.
- Tacer K.F., Bookout A.L., Ding X.S., Kurosu H., John G.B., Wang L., Goetz R., Mohammadi M., Kuro-o M., Mangelsdorf D.J., Kliewer S.A. Research resource: comprehensive expression atlas of the fibroblast growth factor system in adult mouse. Mol Endocrinol 2010; 24(10): 2050–2064, https://doi.org/10.1210/me.2010-0142.
- Bookout A.L., de Groot M.H.M., Owen B.M., Lee S., Gautron L., Lawrence H.L., Ding X.S., Elmquist J.K., Takahashi J.S., Mangelsdorf D.J., Kliewer S.A. FGF21 regulates metabolism and circadian behavior by acting on the nervous system. Nat Med 2013; 19(9): 1147–1152, https://doi.org/10.1038/nm.3249.
- Lee P., Brychta R.J., Linderman J., Smith S., Chen K.Y., Celi F.S. Mild cold exposure modulates fibroblast growth factor 21 (FGF21) diurnal rhythm in humans: relationship between FGF21 levels, lipolysis, and cold-induced thermogenesis. J Clin Endocrinol Metab 2013; 98(1): E98–E102, https://doi.org/10.1210/jc.2012-3107.
- Leng Y., Wang Z., Tsai L.K., Leeds P., Fessler E.B., Wang J., Chuang D.M. FGF-21, a novel metabolic regulator, has a robust neuroprotective role and is markedly elevated in neurons by mood stabilizers. Mol Psychiatr 2015; 20(2): 215–223, https://doi.org/10.1038/mp.2013.192.
- Kuroda M., Muramatsu R., Maedera N., Koyama Y., Hamaguchi M., Fujimura H., Yoshida M., Konishi M., Itoh N., Mochizuki H., Yamashita T. Peripherally derived FGF21 promotes remyelination in the central nervous system. J Clin Invest 2017; 127(9): 3496–3509, https://doi.org/10.1172/JCI94337.
- Amiri M., Braidy N., Aminzadeh M. Protective effects of fibroblast growth factor 21 against amyloid-beta1-42-induced toxicity in SH-SY5Y cells. Neurotox Res 2018; 34(3): 574–583, https://doi.org/10.1007/s12640-018-9914-2.
- Chen J., Hu J., Liu H., Xiong Y., Zou Y.C., Huang W.T., Shao M.J., Wu J.M., Yu L., Wang X.J., Wang X., Lin L. FGF21 protects the blood-brain barrier by upregulating PPARγ via FGFR1/β-klotho after traumatic brain injury. Neurotrauma 2018; 35(17): 2091–2103, https://doi.org/10.1089/neu.2017.5271.
- Jiang Y., Liu N., Wang Q., Yu Z., Lin L., Yuan J., Guo S., Ahn B.J., Wang X.J., Li X., Lo E.H., Sun X., Wang X. Endocrine regulator rFGF21 (recombinant human fibroblast growth factor 21) improves neurological outcomes following focal ischemic stroke of type 2 diabetes mellitus male mice. Stroke 2018; 49(12): 3039–3049, https://doi.org/10.1161/strokeaha.118.022119.
- Restelli L.M., Oettinghaus B., Halliday M., Agca C., Licci M., Sironi L., Savoia C., Hench J., Tolnay M., Neutzner A., Schmidt A., Eckert A., Mallucci G., Scorrano L., Frank S. Neuronal mitochondrial dysfunction activates the integrated stress response to induce fibroblast growth factor 21. Cell Rep 2018; 24(6): 1407–1414, https://doi.org/10.1016/j.celrep.2018.07.023.
- Ruderman N.B., Ross P.S., Berger M., Goodman M.N. Regulation of glucose and ketone-body metabolism in brain of anaesthetized rats. Biochem J 1974; 138(1): 1–10, https://doi.org/10.1042/bj1380001.
- Kwon M.M., O’Dwyer S.M., Baker R.K., Covey S.D., Kieffer T.J. FGF21-mediated improvements in glucose clearance require uncoupling protein 1. Cell Rep 2015; 13(8): 1521–1527, https://doi.org/10.1016/j.celrep.2015.10.021.
- Kharitonenkov A., Wroblewski V.J., Koester A., Chen Y.F., Clutinger C.K., Tigno X.T., Hansen B.C., Shanafelt A.B., Etgen G.J. The metabolic state of diabetic monkeys is regulated by fibroblast growth factor-21. Endocrinology 2007; 148(2): 774–781, https://doi.org/10.1210/en.2006-1168.
- Bostrom P., Wu J., Jedrychowski M.P., Korde A., Ye L., Lo J.C., Rasbach K.A., Bostrom E.A., Choi J.H., Long J.Z., Kajimura S., Zingaretti M.C., Vind B.F., Tu H., Cinti S., Hojlund K., Gygi S.P., Spiegelman B.M. A PGC1-α-dependent myokine that drives brown-fat-like development of white fat and thermogenesis. Nature 2012; 481(7382): 463–468, https://doi.org/10.1038/nature10777.
- Lee P., Linderman J.D., Smith S., Brychta R.J., Wang J., Idelson C., Perron R.M., Werner C.D., Phan G.Q., Kammula U.S., Kebebew E., Pacak K., Chen K.Y., Celi F.S. Irisin and FGF21 are coldinduced endocrine activators of brown fat function in humans. Cell Metab 2014; 19(2): 302–309, https://doi.org/10.1016/j.cmet.2013.12.017.
- Li D.J., Li Y.H., Yuan H.B., Qu L.F., Wang P. The novel exerciseinduced hormone irisin protects against neuronal injury via activation of the Akt and ERK1/2 signaling pathways and contributes to the neuroprotection of physical exercise in cerebral ischemia. Metabolism 2017; 68: 31–42, https://doi.org/10.1016/j.metabol.2016.12.003.
- Asadi Y., Gorjipour F., Behrouzifar S., Vakili A. Irisin peptide protects brain against ischemic injury through reducing apoptosis and enhancing BDNF in a rodent model of stroke. Neurochem Res 2018; 43(8): 1549–1560, https://doi.org/10.1007/s11064-018-2569-9.
- Rao R.R., Long J.Z., White J.P., Svensson K.J., Lou J., Lokurkar I., Jedrychowski M.P., Ruas J.L., Wrann C.D., Lo J.C., Camera D.M., Lachey J., Gygi S., Seehra J., Hawley J.A., Spiegelman B.M. Meteorin-like is a hormone that regulates immune-adipose interactions to increase beige fat thermogenesis. Cell 2014; 157(6): 1279–1291, https://doi.org/10.1016/j.cell.2014.03.065.
- Lan X., Han X., Li Q., Yang Q.W., Wang J. Modulators of microglial activation and polarization after intracerebral haemorrhage. Nat Rev Neurol 2017; 13(7): 420–433, https://doi.org/10.1038/nrneurol.2017.69.
- Danno S., Itoh K., Matsuda T., Fujita J. Decreased expression of mouse Rbm3, a cold-shock protein, in Sertoli cells of cryptorchid testis. Am J Pathol 2000; 156(5): 1685–1692, https://doi.org/10.1016/S0002-9440(10)65039-0.
- Chip S., Zelmer A., Ogunshola O.O., Felderhoff-Mueser U., Nitsch C., Buhrer C., Wellmann S. The RNA-binding protein RBM3 is involved in hypothermia induced neuroprotection. Neurobiol Dis 2011; 43(2): 388–396, https://doi.org/10.1016/j.nbd.2011.04.010.
- Kita H., Carmichael J., Swartz J., Muro S., Wyttenbach A., Matsubara K., Rubinsztein D.C., Kato K. Modulation of polyglutamine-induced cell death by genes identified by expression profiling. Hum Mol Genet 2002; 11(19): 2279–2287, https://doi.org/10.1093/hmg/11.19.2279.
- Yang H.J., Ju F., Guo X.X., Ma S.P., Wang L., Cheng B.F., Zhuang R.J., Zhang B.B., Shi X., Feng Z.W., Wang M. RNA-binding protein RBM3 prevents NO-induced apoptosis in human neuroblastoma cells by modulating p38 signaling and miR-143. Sci Rep 2017; 7: 41738, https://doi.org/10.1038/srep41738.
- Neumar R.W., DeGracia D.J., Konkoly L.L., Khoury J.I., White B.C., Krause G.S. Calpain mediates eukaryotic initiation factor 4G degradation during global brain ischemia. J Cereb Blood Flow Metab 1998; 18(8): 876–881, https://doi.org/10.1097/00004647-199808000-00007.
- de la Vega C.M., Burda J., Nemethova M., Quevedo C., Alcázar A., Martín M.E., Danielisova V., Fando J.L., Salinas M. Possible mechanisms involved in the down-regulation of translation during transient global ischaemia in the rat brain. Biochem J 2001; 357(Pt 3): 819–826, https://doi.org/10.1042/0264-6021:3570819.
- Pilotte J., Dupont-Versteegden E.E., Vanderklish P.W. Widespread regulation of miRNA biogenesis at the Dicer step by the cold-inducible RNA-binding protein, RBM3. PloS One 2011; 6(12): e28446, https://doi.org/10.1371/journal.pone.0028446.
- Peretti D., Bastide A., Radford H., Verity N., Molloy C., Martin M.G., Moreno J.A., Steinert J.R., Smith T., Dinsdale D., Willis A.E., Mallucci G.R. RBM3 mediates structural plasticity and protective effects of cooling in neurodegeneration. Nature 2015; 518(7538): 236–239, https://doi.org/10.1038/nature14142.
- Nishiyama H., Itoh K., Kaneko Y., Kishishita M., Yoshida O., Fujita J. A glycine-rich RNA-binding protein mediating cold-inducible suppression of mammalian cell growth. J Cell Biol 1997; 137(4): 899–908, https://doi.org/10.1083/jcb.137.4.899.
- Li S., Zhang Z., Xue J., Liu A., Zhang H. Cold-inducible RNA binding protein inhibits H2O2-induced apoptosis in rat cortical neurons. Brain Res 2012; 1441: 47–52, https://doi.org/10.1016/j.brainres.2011.12.053.
- Zhang M.J., Li W.J., Niu G.M., Leak R.K., Chen J., Zhang F. ATP induces mild hypothermia in rats but has a strikingly detrimental impact on focal cerebral ischemia. Cereb Blood Flow Metab 2013; 33(1): e1–e10, https://doi.org/10.1038/jcbfm.2012.146.
- Wang G., Zhang J.N., Guo J.K., Cai Y., Sun H.S., Dong K., Wu C.G. Neuroprotective effects of cold-inducible RNA-binding protein during mild hypothermia on traumatic brain injury. Neural Regen Res 2016; 11(5): 771–778, https://doi.org/10.4103/1673-5374.182704.
- Liu J., Xue J., Zhang H., Li S., Liu Y., Xu D., Zou M., Zhang Z., Diao J. Cloning, expression, and purification of cold inducible RNA-binding protein and its neuroprotective mechanism of action. Brain Res 2015; 1597: 189–195, https://doi.org/10.1016/j.brainres.2014.11.061.
- Zhang H.T., Xue J.H., Zhang Z.W., Kong H.B., Liu A.J., Li S.C., Xu D.G. Cold-inducible RNA-binding protein inhibits neuron apoptosis through the suppression of mitochondrial apoptosis. Brain Res 2015; 1622: 474–483, https://doi.org/10.1016/j.brainres.2015.07.004.
- Zhang Q., Wang Y.Z., Zhang W., Chen X., Wang J., Chen J., Luo W. Involvement of cold inducible RNA-binding protein in severe hypoxia-induced growth arrest of neural stem cells in vitro. Mol Neurobiol 2017; 54(3): 2143–2153, https://doi.org/10.1007/s12035-016-9761-1.
- Moreira E.F., Jaworski C.J., Rodriguez I.R. Cloning of a novel member of the reticulon gene family (RTN3): gene structure and chromosomal localization to 11q13. Genomics 1999; 58(1): 73–81, https://doi.org/10.1006/geno.1999.5807.
- Bastide A., Peretti D., Knight J.R.P., Grosso S., Spriggs R.V., Pichon X., Sbarrato T., Roobol A., Roobol J., Vito D., Bushell M., von der Haar T., Smales C.M., Malluccil G.R., Willis A.E. RTN3 is a novel cold-induced protein and mediates neuroprotective effects of RBM3. Curr Biol 2017; 27(5): 638–650, https://doi.org/10.1016/j.cub.2017.01.047.
- Masters C.L., Bateman R., Blennow K., Rowe C.C., Sperling R.A., Cummings J.L. Alzheimer’s disease. Nat Rev Dis Primers 2015; 1: 15056, https://doi.org/10.1038/nrdp.2015.56.
- Deng M., He W., Tan Y., Han H., Hu X., Xia K., Zhang Z., Yan R. Increased expression of reticulon 3 in neurons leads to reduced axonal transport of β site amyloid precursor proteincleaving enzyme 1. Biol Chem 2013; 288(42): 30236–30245, https://doi.org/10.1074/jbc.M113.480079.