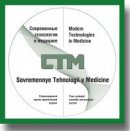
Роль микроРНК в функционировании печени: от биогенеза до терапевтических подходов (обзор)
Молекулярная диагностика на основе панелей малых некодирующих молекул РНК (микроРНК) является новым направлением в современной биомедицине и считается перспективным методом выявления широчайшего спектра патологий на ранней стадии, оценки клинического фенотипа, а также мониторинга течения заболевания, оценки эффективности терапии и риска рецидива заболевания. В настоящее время в ходе изучения нормальных и патологических процессов установлена роль микроРНК как важнейшего эпигенетического регулятора при прогрессировании онкологических заболеваний. Однако работ, посвященных изучению роли микроРНК в функционировании отдельных органов и тканей, а также разработке возможных терапевтических подходов на основе микроРНК, в настоящее время представлено недостаточно. Огромное количество метаболических процессов, происходящих в печени, контролируются микроРНК, что создает огромный потенциал применения их в качестве диагностического маркера, а также мишени для терапевтического вмешательства при метаболических, онкологических и даже вирусных заболеваниях именно этого органа.
Рассмотрены аспекты биологических функций микроРНК в различных типах клеток печени. Представлены как канонические, так и неканонические пути биогенеза, эпигенетической регуляции, опосредованной микроРНК, показана роль микроРНК в межклеточной коммуникации и течении вирусных заболеваний. Описаны потенциал микроРНК как диагностического маркера различных патологий печени, а также одобренные для клинического применения и находящиеся в разработке терапевтические подходы и препараты на основе микроРНК.
- Selbach M., Schwanhäusser B., Thierfelder N., Fang Z., Khanin R., Rajewsky N. Widespread changes in protein synthesis induced by microRNAs. Nature 2008; 455(7209): 58–63, https://doi.org/10.1038/nature07228.
- Kozomara A., Birgaoanu M., Griffiths-Jones S. miRBase: from microRNA sequences to function. Nucleic Acids Res 2019; 47(D1): D155–D162, https://doi.org/10.1093/nar/gky1141.
- Reichholf B., Herzog V.A., Fasching N., Manzenreither R.A., Sowemimo I., Ameres S.L. Time-resolved small RNA sequencing unravels the molecular principles of microRNA homeostasis. Mol Cell 2019; 75(4): 756–768.e7, https://doi.org/10.1016/j.molcel.2019.06.018.
- Stavast C.J., Erkeland S.J. The non-canonical aspects of microRNAs: many roads to gene regulation. Cells 2019; 8(11): 1465, https://doi.org/10.3390/cells8111465.
- Hill M., Tran N. miRNA interplay: mechanisms and consequences in cancer. Dis Model Mech 2021; 14(4): dmm047662, https://doi.org/10.1242/dmm.047662.
- Plawgo K., Raczynska K.D. Context-dependent regulation of gene expression by non-canonical small RNAs. Noncoding RNA 2022; 8(3): 29, https://doi.org/10.3390/ncrna8030029.
- Santovito D., Weber C. Non-canonical features of microRNAs: paradigms emerging from cardiovascular disease. Nat Rev Cardiol 2022; 19(9): 620–638, https://doi.org/10.1038/s41569-022-00680-2.
- Ha M., Kim V.N. Regulation of microRNA biogenesis. Nat Rev Mol Cell Biol 2014; 15(8): 509–524, https://doi.org/10.1038/nrm3838.
- Treiber T., Treiber N., Meister G. Regulation of microRNA biogenesis and its crosstalk with other cellular pathways. Nat Rev Mol Cell Biol. 2019; 20(1): 5–20, https://doi.org/10.1038/s41580-018-0059-1.
- Zapletal D., Taborska E., Pasulka J., Malik R., Kubicek K., Zanova M., Much C., Sebesta M., Buccheri V., Horvat F., Jenickova I., Prochazkova M., Prochazka J., Pinkas M., Novacek J., Joseph D.F., Sedlacek R., Bernecky C., O’Carroll D., Stefl R., Svoboda P. Structural and functional basis of mammalian microRNA biogenesis by Dicer. Mol Cell 2022; 82(21): 4064–4079.e13, https://doi.org/10.1016/j.molcel.2022.10.010.
- Shang R., Lee S., Senavirathne G., Lai E.C. microRNAs in action: biogenesis, function and regulation. Nat Rev Genet 2023, https://doi.org/10.1038/s41576-023-00611-y.
- Londin E., Loher P., Telonis A.G., Quann K., Clark P., Jing Y., Hatzimichael E., Kirino Y., Honda S., Lally M., Ramratnam B., Comstock C.E., Knudsen K.E., Gomella L., Spaeth G.L., Hark L., Katz L.J., Witkiewicz A., Rostami A., Jimenez S.A., Hollingsworth M.A., Yeh J.J., Shaw C.A., McKenzie S.E., Bray P., Nelson P.T., Zupo S., Van Roosbroeck K., Keating M.J., Calin G.A., Yeo C., Jimbo M., Cozzitorto J., Brody J.R., Delgrosso K., Mattick J.S., Fortina P., Rigoutsos I. Analysis of 13 cell types reveals evidence for the expression of numerous novel primate- and tissue-specific microRNAs. Proc Natl Acad Sci U S A 2015; 112(10): E1106–E1115, https://doi.org/10.1073/pnas.1420955112.
- Kuscu C., Kumar P., Kiran M., Su Z., Malik A., Dutta A. tRNA fragments (tRFs) guide Ago to regulate gene expression post-transcriptionally in a Dicer-independent manner. RNA 2018; 24(8): 1093–1105, https://doi.org/10.1261/rna.066126.118.
- Gowda P., Reddy P.H., Kumar S. Deregulated mitochondrial microRNAs in Alzheimer’s disease: focus on synapse and mitochondria. Ageing Res Rev 2022; 73: 101529, https://doi.org/10.1016/j.arr.2021.101529.
- Dambal S., Shah M., Mihelich B., Nonn L. The microRNA-183 cluster: the family that plays together stays together. Nucleic Acids Res 2015; 43(15): 7173–7188, https://doi.org/10.1093/nar/gkv703.
- Ramalingam P., Palanichamy J.K., Singh A., Das P., Bhagat M., Kassab M.A., Sinha S., Chattopadhyay P. Biogenesis of intronic miRNAs located in clusters by independent transcription and alternative splicing. RNA 2014; 20(1): 76–87, https://doi.org/10.1261/rna.041814.113.
- Vilimova M., Pfeffer S. Post-transcriptional regulation of polycistronic microRNAs. Wiley Interdiscip Rev RNA 2023; 14(2): e1749, https://doi.org/10.1002/wrna.1749.
- Donayo A.O., Johnson R.M., Tseng H.W., Izreig S., Gariepy A., Mayya V.K., Wu E., Alam R., Lussier C., Jones R.G., Duchaine T.F. Oncogenic biogenesis of pri-miR-17~92 reveals hierarchy and competition among polycistronic microRNAs. Mol Cell 2019; 75(2): 340–356.e10, https://doi.org/10.1016/j.molcel.2019.05.033.
- Ratnadiwakara M., Engel R., Jarde T., McMurrick P.J., Abud H.E., Änkö M.L. SRSF3 confers selective processing of miR-17-92 cluster to promote tumorigenic properties in colorectal cancer. Cell Biology 2019, https://doi.org/10.1101/667295.
- Gong R., Lv X., Liu F. MiRNA-17 encoded by the miR-17-92 cluster increases the potential for steatosis in hepatoma cells by targeting CYP7A1. Cell Mol Biol Lett 2018; 23: 16, https://doi.org/10.1186/s11658-018-0083-3.
- Najafi-Shoushtari S.H., Kristo F., Li Y., Shioda T., Cohen D.E., Gerszten R.E., Näär A.M. MicroRNA-33 and the SREBP host genes cooperate to control cholesterol homeostasis. Science 2010; 328(5985): 1566–1569, https://doi.org/10.1126/science.1189123.
- Zhang X., Zhao H., Sheng Q., Liu X., You W., Lin H., Liu G. Regulation of microRNA-33, SREBP and ABCA1 genes in a mouse model of high cholesterol. Arch Anim Breed 2021; 64(1): 103–108, https://doi.org/10.5194/aab-64-103-2021.
- Rayner K.J., Suárez Y., Dávalos A., Parathath S., Fitzgerald M.L., Tamehiro N., Fisher E.A., Moore K.J., Fernández-Hernando C. MiR-33 contributes to the regulation of cholesterol homeostasis. Science 2010; 328(5985): 1570–1573, https://doi.org/10.1126/science.1189862.
- Prel A., Dozier C., Combier J.P., Plaza S., Besson A. Evidence that regulation of pri-miRNA/miRNA expression is not a general rule of miPEPs function in humans. Int J Mol Sci 2021; 22(7): 3432, https://doi.org/10.3390/ijms22073432.
- Niu L., Lou F., Sun Y., Sun L., Cai X., Liu Z., Zhou H., Wang H., Wang Z., Bai J., Yin Q., Zhang J., Chen L., Peng D., Xu Z., Gao Y., Tang S., Fan L., Wang H. A micropeptide encoded by lncRNA MIR155HG suppresses autoimmune inflammation via modulating antigen presentation. Sci Adv 2020; 6(21): eaaz2059, https://doi.org/10.1126/sciadv.aaz2059.
- Kim Y.K., Kim B., Kim V.N. Re-evaluation of the roles of DROSHA, Exportin 5, and DICER in microRNA biogenesis. Proc Natl Acad Sci U S A 2016; 113(13): E1881–E1889, https://doi.org/10.1073/pnas.1602532113.
- Park J.E., Heo I., Tian Y., Simanshu D.K., Chang H., Jee D., Patel D.J., Kim V.N. Dicer recognizes the 5’ end of RNA for efficient and accurate processing. Nature 2011; 475(7355): 201–205, https://doi.org/10.1038/nature10198.
- Gu S., Jin L., Zhang Y., Huang Y., Zhang F., Valdmanis P.N., Kay M.A. The loop position of shRNAs and pre-miRNAs is critical for the accuracy of dicer processing in vivo. Cell 2012; 151(4): 900–911, https://doi.org/10.1016/j.cell.2012.09.042.
- Lee Y.Y., Kim H., Kim V.N. Sequence determinant of small RNA production by DICER. Nature 2023; 615(7951): 323–330, https://doi.org/10.1038/s41586-023-05722-4.
- Grimm D., Wang L., Lee J.S., Schürmann N., Gu S., Börner K., Storm T.A., Kay M.A. Argonaute proteins are key determinants of RNAi efficacy, toxicity, and persistence in the adult mouse liver. J Clin Invest 2010; 120(9): 3106–3119, https://doi.org/10.1172/jci43565.
- Valdmanis P.N., Gu S., Schüermann N., Sethupathy P., Grimm D., Kay M.A. Expression determinants of mammalian argonaute proteins in mediating gene silencing. Nucleic Acids Res 2012; 40(8): 3704–3713, https://doi.org/10.1093/nar/gkr1274.
- Wu J., Yang J., Cho W.C., Zheng Y. Argonaute proteins: structural features, functions and emerging roles. J Adv Res 2020; 24: 317–324, https://doi.org/10.1016/j.jare.2020.04.017.
- Meijer H.A., Smith E.M., Bushell M. Regulation of miRNA strand selection: follow the leader? Biochem Soc Trans 2014; 42(4): 1135–1140, https://doi.org/10.1042/bst20140142.
- Liao Y., Jung S.H., Kim T. A-to-I RNA editing as a tuner of noncoding RNAs in cancer. Cancer Lett 2020; 494: 88–93, https://doi.org/10.1016/j.canlet.2020.08.004.
- Cloonan N., Wani S., Xu Q., Gu J., Lea K., Heater S., Barbacioru C., Steptoe A.L., Martin H.C., Nourbakhsh E., Krishnan K., Gardiner B., Wang X., Nones K., Steen J.A., Matigian N.A., Wood D.L., Kassahn K.S., Waddell N., Shepherd J., Lee C., Ichikawa J., McKernan K., Bramlett K., Kuersten S., Grimmond S.M. MicroRNAs and their isomiRs function cooperatively to target common biological pathways. Genome Biol 2011; 12(12): R126, https://doi.org/10.1186/gb-2011-12-12-r126.
- Tan G.C., Chan E., Molnar A., Sarkar R., Alexieva D., Isa I.M., Robinson S., Zhang S., Ellis P., Langford C.F., Guillot P.V., Chandrashekran A., Fisk N.M., Castellano L., Meister G., Winston R.M., Cui W., Baulcombe D., Dibb N.J. 5′ isomiR variation is of functional and evolutionary importance. Nucleic Acids Res 2014; 42(14): 9424–9435, https://doi.org/10.1093/nar/gku656.
- Dika E., Broseghini E., Porcellini E., Lambertini M., Riefolo M., Durante G., Loher P., Roncarati R., Bassi C., Misciali C., Negrini M., Rigoutsos I., Londin E., Patrizi A., Ferracin M. Unraveling the role of microRNA/isomiR network in multiple primary melanoma pathogenesis. Cell Death Dis 2021; 12(5): 473, https://doi.org/10.1038/s41419-021-03764-y.
- Park S., Yang H.D., Seo J.W., Nam J.W., Nam S.W. hnRNPC induces isoform shifts in miR-21-5p leading to cancer development. Exp Mol Med 2022; 54(6): 812–824, https://doi.org/10.1038/s12276-022-00792-2.
- Tomasello L., Distefano R., Nigita G., Croce C.M. The microRNA family gets wider: the isomiRs classification and role. Front Cell Dev Biol 2021; 9: 668648, https://doi.org/10.3389/fcell.2021.668648.
- Schamberger A., Orbán T.I. 3′ isomiR species and DNA contamination influence reliable quantification of microRNAs by stem-loop quantitative PCR. PLoS One 2014; 9(8): e106315, https://doi.org/10.1371/journal.pone.0106315.
- Zhiyanov A., Nersisyan S., Tonevitsky A. Hairpin sequence and structure is associated with features of isomiR biogenesis. RNA Biol 2021; 18(sup1): 430–438, https://doi.org/10.1080/15476286.2021.1952759.
- McGeary S.E., Bisaria N., Pham T.M., Wang P.Y., Bartel D.P. MicroRNA 3′-compensatory pairing occurs through two binding modes, with affinity shaped by nucleotide identity and position. Elife 2022; 11: e69803, https://doi.org/10.7554/elife.69803.
- Luna J.M., Barajas J.M., Teng K.Y., Sun H.L., Moore M.J., Rice C.M., Darnell R.B., Ghoshal K. Argonaute CLIP defines a deregulated miR-122-bound transcriptome that correlates with patient survival in human liver cancer. Mol Cell 2017; 67(3): 400–410.e7, https://doi.org/10.1016/j.molcel.2017.06.025.
- Ghini F., Rubolino C., Climent M., Simeone I., Marzi M.J., Nicassio F. Endogenous transcripts control miRNA levels and activity in mammalian cells by target-directed miRNA degradation. Nat Commun 2018; 9(1): 3119, https://doi.org/10.1038/s41467-018-05182-9.
- Rani V., Sengar R.S. Biogenesis and mechanisms of microRNA-mediated gene regulation. Biotechnol Bioeng 2022; 119(3): 685–692, https://doi.org/10.1002/bit.28029.
- Kilikevicius A., Meister G., Corey D.R. Reexamining assumptions about miRNA-guided gene silencing. Nucleic Acids Res 2022; 50(2): 617–634, https://doi.org/10.1093/nar/gkab1256.
- Wahid F., Shehzad A., Khan T., Kim Y.Y. MicroRNAs: synthesis, mechanism, function, and recent clinical trials. Biochim Biophys Acta 2010; 1803(11): 1231–1243, https://doi.org/10.1016/j.bbamcr.2010.06.013.
- Naeli P., Winter T., Hackett A.P., Alboushi L., Jafarnejad S.M. The intricate balance between microRNA-induced mRNA decay and translational repression. FEBS J 2023; 290(10): 2508–2524, https://doi.org/10.1111/febs.16422.
- Fukao A., Mishima Y., Takizawa N., Oka S., Imataka H., Pelletier J., Sonenberg N., Thoma C., Fujiwara T. MicroRNAs trigger dissociation of eIF4AI and eIF4AII from target mRNAs in humans. Mol Cell 2014; 56(1): 79–89, https://doi.org/10.1016/j.molcel.2014.09.005.
- Luo Y., Na Z., Slavoff S.A. P-bodies: composition, properties, and functions. Biochemistry 2018; 57(17): 2424–2431, https://doi.org/10.1021/acs.biochem.7b01162.
- Ivanov P., Kedersha N., Anderson P. Stress granules and processing bodies in translational control. Cold Spring Harb Perspect Biol 2019; 11(5): a032813, https://doi.org/10.1101/cshperspect.a032813.
- Chipman L.B., Pasquinelli A.E. miRNA targeting: growing beyond the seed. Trends Genet 2019; 35(3): 215–222, https://doi.org/10.1016/j.tig.2018.12.005.
- Baronti L., Guzzetti I., Ebrahimi P., Friebe Sandoz S., Steiner E., Schlagnitweit J., Fromm B., Silva L., Fontana C., Chen A.A., Petzold K. Base-pair conformational switch modulates miR-34a targeting of Sirt1 mRNA. Nature 2020; 583(7814): 139–144, https://doi.org/10.1038/s41586-020-2336-3.
- Peter D., Ruscica V., Bawankar P., Weber R., Helms S., Valkov E., Igreja C., Izaurralde E. Molecular basis for GIGYF–Me31B complex assembly in 4EHP-mediated translational repression. Genes Dev 2019; 33(19–20): 1355–1360, https://doi.org/10.1101/gad.329219.119.
- Chowdhury A., Mukhopadhyay J., Tharun S. The decapping activator Lsm1p-7p–Pat1p complex has the intrinsic ability to distinguish between oligoadenylated and polyadenylated RNAs. RNA 2007; 13(7): 998–1016, https://doi.org/10.1261/rna.502507.
- Marques T.M., Gama-Carvalho M. Network approaches to study endogenous RNA competition and its impact on tissue-specific microRNA functions. Biomolecules 2022; 12(2): 332, https://doi.org/10.3390/biom12020332.
- Yu J., Ryan D.G., Getsios S., Oliveira-Fernandes M., Fatima A., Lavker R.M. MicroRNA-184 antagonizes microRNA-205 to maintain SHIP2 levels in epithelia. Proc Natl Acad Sci U S A 2008; 105(49): 19300–19305, https://doi.org/10.1073/pnas.0803992105.
- Rinck A., Preusse M., Laggerbauer B., Lickert H., Engelhardt S., Theis F.J. The human transcriptome is enriched for miRNA-binding sites located in cooperativity-permitting distance. RNA Biol 2013; 10(7): 1125–1135, https://doi.org/10.4161/rna.24955.
- Briskin D., Wang P.Y., Bartel D.P. The biochemical basis for the cooperative action of microRNAs. Proc Natl Acad Sci U S A 2020; 117(30): 17764–17774, https://doi.org/10.1073/pnas.1920404117.
- Sheu-Gruttadauria J., Pawlica P., Klum S.M., Wang S., Yario T.A., Schirle Oakdale N.T., Steitz J.A., MacRae I.J. Structural basis for target-directed microRNA degradation. Mol Cell 2019; 75(6): 1243–1255.e7, https://doi.org/10.1016/j.molcel.2019.06.019.
- Shi C.Y., Kingston E.R., Kleaveland B., Lin D.H., Stubna M.W., Bartel D.P. The ZSWIM8 ubiquitin ligase mediates target-directed microRNA degradation. Science 2020; 370(6523): eabc9359, https://doi.org/10.1126/science.abc9359.
- Han J., Mendell J.T. MicroRNA turnover: a tale of tailing, trimming, and targets. Trends Biochem Sci 2023; 48(1): 26–39, https://doi.org/10.1016/j.tibs.2022.06.005.
- Park J.H., Shin S.Y., Shin C. Non-canonical targets destabilize microRNAs in human Argonautes. Nucleic Acids Res 2017; 45(4): 1569–1583, https://doi.org/10.1093/nar/gkx029.
- Becker W.R., Ober-Reynolds B., Jouravleva K., Jolly S.M., Zamore P.D., Greenleaf W.J. High-throughput analysis reveals rules for target RNA binding and cleavage by AGO2. Mol Cell 2019; 75(4): 741–755.e11, https://doi.org/10.1016/j.molcel.2019.06.012.
- Komatsu S., Kitai H., Suzuki H.I. Network regulation of microRNA biogenesis and target interaction. Cells 2023; 12(2): 306, https://doi.org/10.3390/cells12020306.
- Khatun S., Alam A., Shoombuatong W., Mollah N.H., Kurata H., Hasan M. Recent development of bioinformatics tools for microRNA target prediction. CMC 2022; 29(5): 865–880, https://doi.org/10.2174/0929867328666210804090224.
- Kariuki D., Asam K., Aouizerat B.E., Lewis K.A., Florez J.C., Flowers E. Review of databases for experimentally validated human microRNA–mRNA interactions. Database (Oxford) 2023; 2023: baad014, https://doi.org/10.1093/database/baad014.
- Dragomir M.P., Knutsen E., Calin G.A. Classical and noncanonical functions of miRNAs in cancers. Trends Genet 2022; 38(4): 379–394, https://doi.org/10.1016/j.tig.2021.10.002.
- Ni W.J., Leng X.M. miRNA-dependent activation of mRNA translation. Microrna 2016; 5(2): 83–86, https://doi.org/10.2174/2211536605666160825151201.
- Wakiyama M., Ogami K., Iwaoka R., Aoki K., Hoshino S. Micro RNP-mediated translational activation of nonadenylated mRNAs in a mammalian cell-free system. Genes Cells 2018; 23(5): 332–344, https://doi.org/10.1111/gtc.12580.
- Barman B., Bhattacharyya S.N. mRNA targeting to endoplasmic reticulum precedes ago protein interaction and microRNA (miRNA)-mediated translation repression in mammalian cells. J Biol Chem 2015; 290(41): 24650–24656, https://doi.org/10.1074/jbc.c115.661868.
- Nishi K., Takahashi T., Suzawa M., Miyakawa T., Nagasawa T., Ming Y., Tanokura M., Ui-Tei K. Control of the localization and function of a miRNA silencing component TNRC6A by Argonaute protein. Nucleic Acids Res, 2015; 43(20): 9856–9873, https://doi.org/10.1093/nar/gkv1026.
- Detzer A., Engel C., Wünsche W., Sczakiel G. Cell stress is related to re-localization of Argonaute 2 and to decreased RNA interference in human cells. Nucleic Acids Res 2011; 39(7): 2727–2741, https://doi.org/10.1093/nar/gkq1216.
- Bose M., Barman B., Goswami A., Bhattacharyya S.N. Spatiotemporal uncoupling of microRNA-mediated translational repression and target RNA degradation controls microRNP recycling in mammalian cells. Mol Cell Biol 2017; 37(4): e00464–16, https://doi.org/10.1128/mcb.00464-16.
- Gibbings D.J., Ciaudo C., Erhardt M., Voinnet O. Multivesicular bodies associate with components of miRNA effector complexes and modulate miRNA activity. Nat Cell Biol 2009; 11(9): 1143–1149, https://doi.org/10.1038/ncb1929.
- Bian Z., Li L.M., Tang R., Hou D.X., Chen X., Zhang C.Y., Zen K. Identification of mouse liver mitochondria-associated miRNAs and their potential biological functions. Cell Res 2010; 20(9): 1076–1078, https://doi.org/10.1038/cr.2010.119.
- Das S., Ferlito M., Kent O.A., Fox-Talbot K., Wang R., Liu D., Raghavachari N., Yang Y., Wheelan S.J., Murphy E., Steenbergen C. Nuclear miRNA regulates the mitochondrial genome in the heart. Circ Res 2012; 110(12): 1596–1603, https://doi.org/10.1161/circresaha.112.267732.
- Meister G., Landthaler M., Patkaniowska A., Dorsett Y., Teng G., Tuschl T. Human Argonaute2 mediates RNA cleavage targeted by miRNAs and siRNAs. Mol Cell 2004; 15(2): 185–197, https://doi.org/10.1016/j.molcel.2004.07.007.
- Hwang H.W., Wentzel E.A., Mendell J.T. A hexanucleotide element directs microRNA nuclear import. Science 2007; 315(5808): 97–100, https://doi.org/10.1126/science.1136235.
- Doyle M., Badertscher L., Jaskiewicz L., Güttinger S., Jurado S., Hugenschmidt T., Kutay U., Filipowicz W. The double-stranded RNA binding domain of human Dicer functions as a nuclear localization signal. RNA 2013; 19(9): 1238–1252, https://doi.org/10.1261/rna.039255.113.
- Nishi K., Nishi A., Nagasawa T., Ui-Tei K. Human TNRC6A is an Argonaute-navigator protein for microRNA-mediated gene silencing in the nucleus. RNA 2013; 19(1): 17–35, https://doi.org/10.1261/rna.034769.112.
- Ando Y., Tomaru Y., Morinaga A., Burroughs A.M., Kawaji H., Kubosaki A., Kimura R., Tagata M., Ino Y., Hirano H., Chiba J., Suzuki H., Carninci P., Hayashizaki Y. Nuclear pore complex protein mediated nuclear localization of dicer protein in human cells. PLoS One 2011; 6(8): e23385, https://doi.org/10.1371/journal.pone.0023385.
- Hu X., Li Y., Zhang T., Li L., Chen S., Wu X., Li H., Qi B., Chen Z. Phosphorylation of Ago2 is required for its role in DNA double-strand break repair. J Genet Genomics 2021; 48(4): 333–340, https://doi.org/10.1016/j.jgg.2021.03.011.
- Carissimi C., Laudadio I., Cipolletta E., Gioiosa S., Mihailovich M., Bonaldi T., Macino G., Fulci V. Argonaute2 cooperates with SWI/SNF complex to determine nucleosome occupancy at human Transcription Start Sites. Nucleic Acids Res 2015; 43(3): 1498–1512, https://doi.org/10.1093/nar/gku1387.
- Bieluszewski T., Prakash S., Roulé T., Wagner D. The role and activity of SWI/SNF chromatin remodelers. Annu Rev Plant Biol 2023; 74: 139–163, https://doi.org/10.1146/annurev-arplant-102820-093218.
- Huang V., Zheng J., Qi Z., Wang J., Place R.F., Yu J., Li H., Li L.C. Ago1 interacts with RNA polymerase II and binds to the promoters of actively transcribed genes in human cancer cells. PLoS Genet 2013; 9(9): e1003821, https://doi.org/10.1371/journal.pgen.1003821.
- Matsui M., Li L., Janowski B.A., Corey D.R. Reduced expression of Argonaute 1, Argonaute 2 and TRBP changes levels and intracellular distribution of RNAi factors. Sci Rep 2015; 5(1): 12855, https://doi.org/10.1038/srep12855.
- Ameyar-Zazoua M., Rachez C., Souidi M., Robin P., Fritsch L., Young R., Morozova N., Fenouil R., Descostes N., Andrau J.C., Mathieu J., Hamiche A., Ait-Si-Ali S., Muchardt C., Batsché E., Harel-Bellan A. Argonaute proteins couple chromatin silencing to alternative splicing. Nat Struct Mol Biol 2012; 19(10): 998–1004, https://doi.org/10.1038/nsmb.2373.
- Pitchiaya S., Heinicke L.A., Park J.I., Cameron E.L., Walter N.G. Resolving subcellular miRNA trafficking and turnover at single-molecule resolution. Cell Rep 2017; 19(3): 630–642, https://doi.org/10.1016/j.celrep.2017.03.075.
- Yao Q., Chen Y., Zhou X. The roles of microRNAs in epigenetic regulation. Curr Opin Chem Biol 2019; 51: 11–17, https://doi.org/10.1016/j.cbpa.2019.01.024.
- Zhang T., Yang Z., Kusumanchi P., Han S., Liangpunsakul S. Critical role of microRNA-21 in the pathogenesis of liver diseases. Front Med (Lausanne) 2020; 7: 7, https://doi.org/10.3389/fmed.2020.00007.
- Wang B., Majumder S., Nuovo G., Kutay H., Volinia S., Patel T., Schmittgen T.D., Croce C., Ghoshal K., Jacob S.T. Role of microRNA-155 at early stages of hepatocarcinogenesis induced by choline-deficient and amino acid-defined diet in C57BL/6 mice. Hepatology 2009; 50(4): 1152–1161, https://doi.org/10.1002/hep.23100.
- Liang Y., Lu Q., Li W., Zhang D., Zhang F., Zou Q., Chen L., Tong Y., Liu M., Wang S., Li W., Ren X., Xu P., Yang Z., Dong S., Zhang B., Huang Y., Li D., Wang H., Yu W. Reactivation of tumour suppressor in breast cancer by enhancer switching through NamiRNA network. Nucleic Acids Res 2021; 49(15): 8556–8572, https://doi.org/10.1093/nar/gkab626.
- Xiao M., Li J., Li W., Wang Y., Wu F., Xi Y., Zhang L., Ding C., Luo H., Li Y., Peng L., Zhao L., Peng S., Xiao Y., Dong S., Cao J., Yu W. MicroRNAs activate gene transcription epigenetically as an enhancer trigger. RNA Biol 2017; 14(10): 1326–1334, https://doi.org/10.1080/15476286.2015.1112487.
- Liu S., He X., Di Y., Li Q., Li F., Ma Y., Chen L., Gao Y., Xu J., Yang S., Xu L., Corpe C., Ling Y., Zhang X., Xu J., Yu W., Wang J. NamiRNA-enhancer network of miR-492 activates the NR2C1-TGF-β/Smad3 pathway to promote epithelial-mesenchymal transition of pancreatic cancer. Carcinogenesis 2023; 44(2): 153–165, https://doi.org/10.1093/carcin/bgac102.
- Zou S., Rao Y., Chen W. miR-885-5p plays an accomplice role in liver cancer by instigating TIGAR expression via targeting its promoter. Biotechnol Appl Biochem 2019; 66(5): 763–771, https://doi.org/10.1002/bab.1767.
- Matsui M., Chu Y., Zhang H., Gagnon K.T., Shaikh S., Kuchimanchi S., Manoharan M., Corey D.R., Janowski B.A. Promoter RNA links transcriptional regulation of inflammatory pathway genes. Nucleic Acids Res 2013; 41(22): 10086–10109, https://doi.org/10.1093/nar/gkt777.
- Yang H., Xuefeng Y., Shandong W., Jianhua X. COX-2 in liver fibrosis. Clin Chim Acta 2020; 506: 196–203, https://doi.org/10.1016/j.cca.2020.03.024.
- Martín-Sanz P., Casado M., Boscá L. Cyclooxygenase 2 in liver dysfunction and carcinogenesis: facts and perspectives. World J Gastroenterol 2017; 23(20): 3572–3580, https://doi.org/10.3748/wjg.v23.i20.3572.
- Liu H., Lei C., He Q., Pan Z., Xiao D., Tao Y. Nuclear functions of mammalian MicroRNAs in gene regulation, immunity and cancer. Mol Cancer 2018; 17(1): 64, https://doi.org/10.1186/s12943-018-0765-5.
- Miao L., Yao H., Li C., Pu M., Yao X., Yang H., Qi X., Ren J., Wang Y. A dual inhibition: microRNA-552 suppresses both transcription and translation of cytochrome P450 2E1. Biochim Biophys Acta 2016; 1859(4): 650–662, https://doi.org/10.1016/j.bbagrm.2016.02.016.
- Zhang Y., Fan M., Zhang X., Huang F., Wu K., Zhang J., Liu J., Huang Z., Luo H., Tao L., Zhang H. Cellular microRNAs up-regulate transcription via interaction with promoter TATA-box motifs. RNA 2014; 20(12): 1878–1889, https://doi.org/10.1261/rna.045633.114.
- Zhang Y., Liu W., Chen Y., Liu J., Wu K., Su L., Zhang W., Jiang Y., Zhang X., Zhang Y., Liu C., Tao L., Liu B., Zhang H. A cellular microRNA facilitates regulatory T lymphocyte development by targeting the FOXP3 promoter TATA-box motif. J Immunol 2018; 200(3): 1053–1063, https://doi.org/10.4049/jimmunol.1700196.
- Kurt A.S., Strobl K., Ruiz P., Osborn G., Chester T., Dawson L., Warwas K.M., Grey E.H., Mastoridis S., Kodela E., Safinia N., Sanchez-Fueyo A., Martinez-Llordella M. IL-2 availability regulates the tissue specific phenotype of murine intra-hepatic Tregs. Front Immunol 2022; 13: 1040031, https://doi.org/10.3389/fimmu.2022.1040031.
- Tang S., Chen Y., Feng S., Yi T., Liu X., Li Q., Liu Z., Zhu C., Hu J., Yu X., Wang M., Cao G., Tang H., Bie C., Ma F., Tang H., Du G., Huang J. MiR-483-5p promotes IGF-II transcription and is associated with poor prognosis of hepatocellular carcinoma. Oncotarget 2017; 8(59): 99871–99888, https://doi.org/10.18632/oncotarget.21737.
- Lu J., Guo J., Liu J., Mao X., Xu K. Long non-coding RNA MALAT1: a key player in liver diseases. Front Med (Lausanne) 2022; 8: 734643, https://doi.org/10.3389/fmed.2021.734643.
- Leucci E., Patella F., Waage J., Holmstrøm K., Lindow M., Porse B., Kauppinen S., Lund A.H. microRNA-9 targets the long non-coding RNA MALAT1 for degradation in the nucleus. Sci Rep 2013; 3: 2535, https://doi.org/10.1038/srep02535.
- Tang R., Li L., Zhu D., Hou D., Cao T., Gu H., Zhang J., Chen J., Zhang C.Y., Zen K. Mouse miRNA-709 directly regulates miRNA-15a/16-1 biogenesis at the posttranscriptional level in the nucleus: evidence for a microRNA hierarchy system. Cell Res 2012; 22(3): 504–515, https://doi.org/10.1038/cr.2011.137.
- Wang D., Sun X., Wei Y., Liang H., Yuan M., Jin F., Chen X., Liu Y., Zhang C.Y., Li L., Zen K. Nuclear miR-122 directly regulates the biogenesis of cell survival oncomiR miR-21 at the posttranscriptional level. Nucleic Acids Res 2018; 46(4): 2012–2029, https://doi.org/10.1093/nar/gkx1254.
- Chen Y., Xiao J., Zhang X., Bian X. MicroRNAs as key mediators of hepatic detoxification. Toxicology 2016; 368–369: 80–90, https://doi.org/10.1016/j.tox.2016.08.005.
- Dalgaard L.T., Sørensen A.E., Hardikar A.A., Joglekar M.V. The microRNA-29 family: role in metabolism and metabolic disease. Am J Physiol Cell Physiol 2022; 323(2): C367–C377, https://doi.org/10.1152/ajpcell.00051.2022.
- Agbu P., Carthew R.W. MicroRNA-mediated regulation of glucose and lipid metabolism. Nat Rev Mol Cell Biol 2021; 22(6): 425–438, https://doi.org/10.1038/s41580-021-00354-w.
- Fang Z., Dou G., Wang L. MicroRNAs in the pathogenesis of nonalcoholic fatty liver disease. Int J Biol Sci 2021; 17(7): 1851–1863, https://doi.org/10.7150/ijbs.59588.
- Wang X., He Y., Mackowiak B., Gao B. MicroRNAs as regulators, biomarkers and therapeutic targets in liver diseases. Gut 2021; 70(4): 784–795, https://doi.org/10.1136/gutjnl-2020-322526.
- Citrin K.M., Fernández-Hernando C., Suárez Y. MicroRNA regulation of cholesterol metabolism. Ann N Y Acad Sci 2021; 1495(1): 55–77, https://doi.org/10.1111/nyas.14566.
- Al-Gazally M.E., Khan R., Imran M., Ramírez-Coronel A.A., Alshahrani S.H., Altalbawy F.M.A., Turki Jalil A., Romero-Parra R.M., Zabibah R.S., Shahid Iqbal M., Karampoor S., Mirzaei R. The role and mechanism of action of microRNA-122 in cancer: focusing on the liver. Int Immunopharmacol 2023; 123: 110713, https://doi.org/10.1016/j.intimp.2023.110713.
- Goncalves B.d.S., Meadows A., Pereira D.G., Puri R., Pillai S.S. Insight into the inter-organ crosstalk and prognostic role of liver-derived micrornas in metabolic disease progression. Biomedicines 2023; 11(6): 1597, https://doi.org/10.3390/biomedicines11061597.
- Willeit P., Skroblin P., Kiechl S., Fernández-Hernando C., Mayr M. Liver microRNAs: potential mediators and biomarkers for metabolic and cardiovascular disease? Eur Heart J 2016; 37(43): 3260–3266, https://doi.org/10.1093/eurheartj/ehw146.
- Stojkovic S., Koller L., Sulzgruber P., Hülsmann M., Huber K., Mayr M., Hengstenberg C., Wojta J., Niessner A. Liver-specific microRNA-122 as prognostic biomarker in patients with chronic systolic heart failure. Int J Cardiol 2020; 303: 80–85, https://doi.org/10.1016/j.ijcard.2019.11.090.
- Marques F.Z., Vizi D., Khammy O., Mariani J.A., Kaye D.M. The transcardiac gradient of cardio-microRNAs in the failing heart: transcardiac microRNAs in heart failure. Eur J Heart Fail 2016; 18(8): 1000–1008, https://doi.org/10.1002/ejhf.517.
- Shi Y., Zhang Z., Yin Q., Fu C., Barszczyk A., Zhang X., Wang J., Yang D. Cardiac-specific overexpression of miR-122 induces mitochondria-dependent cardiomyocyte apoptosis and promotes heart failure by inhibiting Hand2. J Cell Mol Med 2021; 25(11): 5326–5334, https://doi.org/10.1111/jcmm.16544.
- van der Meer A.J., Farid W.R., Sonneveld M.J., de Ruiter P.E., Boonstra A., van Vuuren A.J., Verheij J., Hansen B.E., de Knegt R.J., van der Laan L.J., Janssen H.L. Sensitive detection of hepatocellular injury in chronic hepatitis C patients with circulating hepatocyte-derived microRNA-122. J Viral Hepat 2013; 20(3): 158–166, https://doi.org/10.1111/jvh.12001.
- Roderburg C., Trautwein C. Cell-specific functions of miRNA in the liver. J Hepatol 2017; 66(3): 655–656, https://doi.org/10.1016/j.jhep.2016.09.015.
- Zhang Y., Tan Y.Y., Chen P.P., Xu H., Xie S.J., Xu S.J., Li B., Li J.H., Liu S., Yang J.H., Zhou H., Qu L.H. Genome-wide identification of microRNA targets reveals positive regulation of the Hippo pathway by miR-122 during liver development. Cell Death Dis 2021; 12(12): 1161, https://doi.org/10.1038/s41419-021-04436-7.
- Gamazon E.R., Innocenti F., Wei R., Wang L., Zhang M., Mirkov S., Ramírez J., Huang R.S., Cox N.J., Ratain M.J., Liu W. A genome-wide integrative study of microRNAs in human liver. BMC Genomics 2013; 14(1): 395, https://doi.org/10.1186/1471-2164-14-395.
- Torres J.L., Novo-Veleiro I., Manzanedo L., Alvela-Suárez L., Macías R., Laso F.J., Marcos M. Role of microRNAs in alcohol-induced liver disorders and non-alcoholic fatty liver disease. World J Gastroenterol 2018; 24(36): 4104–4118, https://doi.org/10.3748/wjg.v24.i36.4104.
- Lin X., Chen L., Li H., Liu Y., Guan Y., Li X., Jia Z., Lin X., Jia J., Sun Y., Xiao D. miR-155 accelerates proliferation of mouse hepatocytes during liver regeneration by directly targeting SOCS1. Am J Physiol Gastrointest Liver Physiol 2018; 315(4): G443–G453, https://doi.org/10.1152/ajpgi.00072.2018.
- Kim J.H., Lee C.H., Lee S.W. Exosomal transmission of MicroRNA from HCV replicating cells stimulates transdifferentiation in hepatic stellate cells. Mol Ther Nucleic Acids 2019; 14: 483–497, https://doi.org/10.1016/j.omtn.2019.01.006.
- Wang H., Wang Z., Wang Y., Li X., Yang W., Wei S., Shi C., Qiu J., Ni M., Rao J., Cheng F. miRNA-130b-5p promotes hepatic stellate cell activation and the development of liver fibrosis by suppressing SIRT4 expression. J Cell Mol Med 2021; 25(15): 7381–7394, https://doi.org/10.1111/jcmm.16766.
- Wei J., Feng L., Li Z., Xu G., Fan X. MicroRNA-21 activates hepatic stellate cells via PTEN/Akt signaling. Biomed Pharmacother 2013; 67(5): 387–392, https://doi.org/10.1016/j.biopha.2013.03.014.
- Murakami Y., Toyoda H., Tanaka M., Kuroda M., Harada Y., Matsuda F., Tajima A., Kosaka N., Ochiya T., Shimotohno K. The progression of liver fibrosis is related with overexpression of the miR-199 and 200 families. PLoS One 2011; 6(1): e16081, https://doi.org/10.1371/journal.pone.0016081.
- Wu J., Huang J., Kuang S., Chen J., Li X., Chen B., Wang J., Cheng D., Shuai X. Synergistic microRNA therapy in liver fibrotic rat using MRI-visible nanocarrier targeting hepatic stellate cells. Adv Sci (Weinh) 2019; 6(5): 1801809, https://doi.org/10.1002/advs.201801809.
- O’Reilly S. MicroRNAs in fibrosis: opportunities and challenges. Arthritis Res Ther 2016; 18: 11, https://doi.org/10.1186/s13075-016-0929-x.
- Lakner A.M., Steuerwald N.M., Walling T.L., Ghosh S., Li T., McKillop I.H., Russo M.W., Bonkovsky H.L., Schrum L.W. Inhibitory effects of microRNA 19b in hepatic stellate cell-mediated fibrogenesis. Hepatology 2012; 56(1): 300–310, https://doi.org/10.1002/hep.25613.
- Zhou Y., Zhang L., Ji H., Lu X., Xia J., Li L., Chen F., Bu H., Shi Y. MiR-17~92 ablation impairs liver regeneration in an estrogen-dependent manner. J Cell Mol Med 2016; 20(5): 939–948, https://doi.org/10.1111/jcmm.12782.
- Bala S., Csak T., Kodys K., Catalano D., Ambade A., Furi I., Lowe P., Cho Y., Iracheta-Vellve A., Szabo G. Alcohol-induced miR-155 and HDAC11 inhibit negative regulators of the TLR4 pathway and lead to increased LPS responsiveness of Kupffer cells in alcoholic liver disease. J Leukoc Biol 2017; 102(2): 487–498, https://doi.org/10.1189/jlb.3a0716-310r.
- Ye D., Zhang T., Lou G., Liu Y. Role of miR-223 in the pathophysiology of liver diseases. Exp Mol Med 2018; 50(9): 1–12, https://doi.org/10.1038/s12276-018-0153-7.
- He Y., Feng D., Li M., Gao Y., Ramirez T., Cao H., Kim S.J., Yang Y., Cai Y., Ju C., Wang H., Li J., Gao B. Hepatic mitochondrial DNA/Toll-like receptor 9/MicroRNA-223 forms a negative feedback loop to limit neutrophil overactivation and acetaminophen hepatotoxicity in mice. Hepatology 2017; 66(1): 220–234, https://doi.org/10.1002/hep.29153.
- Catanesi M., d’Angelo M., Tupone M.G., Benedetti E., Giordano A., Castelli V., Cimini A. MicroRNAs dysregulation and mitochondrial dysfunction in neurodegenerative diseases. Int J Mol Sci 2020; 21(17): 5986, https://doi.org/10.3390/ijms21175986.
- Zhao X., Xue X., Wang C., Wang J., Peng C., Li Y. Emerging roles of Sirtuins in alleviating alcoholic liver disease: a comprehensive review. Int Immunopharmacol 2022; 108: 108712, https://doi.org/10.1016/j.intimp.2022.108712.
- Zang M., Gao B. SIRT6: therapeutic target for nonalcoholic fatty liver disease. Trends Endocrinol Metab 2022; 33(12): 801–803, https://doi.org/10.1016/j.tem.2022.10.004.
- de Gregorio E., Colell A., Morales A., Marí M. Relevance of SIRT1-NF-κB axis as therapeutic target to ameliorate inflammation in liver disease. Int J Mol Sci 2020; 21(11): 3858, https://doi.org/10.3390/ijms21113858.
- Zhang J., Xiang H., Liu J., Chen Y., He R.R., Liu B. Mitochondrial Sirtuin 3: new emerging biological function and therapeutic target. Theranostics 2020; 10(18): 8315–8342, https://doi.org/10.7150/thno.45922.
- Rottiers V., Najafi-Shoushtari S.H., Kristo F., Gurumurthy S., Zhong L., Li Y., Cohen D.E., Gerszten R.E., Bardeesy N., Mostoslavsky R., Näär A.M. MicroRNAs in metabolism and metabolic diseases. Cold Spring Harb Symp Quant Biol 2011; 76: 225–233, https://doi.org/10.1101/sqb.2011.76.011049.
- Wang L., Sun M., Cao Y., Ma L., Shen Y., Velikanova A.A., Li X., Sun C., Zhao Y. miR-34a regulates lipid metabolism by targeting SIRT1 in non-alcoholic fatty liver disease with iron overload. Arch Biochem Biophys 2020; 695: 108642, https://doi.org/10.1016/j.abb.2020.108642.
- Song J.J., Yang M., Liu Y., Song J.W., Wang J., Chi H.J., Liu X.Y., Zuo K., Yang X.C., Zhong J.C. MicroRNA-122 aggravates angiotensin II-mediated apoptosis and autophagy imbalance in rat aortic adventitial fibroblasts via the modulation of SIRT6-elabela-ACE2 signaling. Eur J Pharmacol 2020; 883: 173374, https://doi.org/10.1016/j.ejphar.2020.173374.
- Elhanati S., Ben-Hamo R., Kanfi Y., Varvak A., Glazz R., Lerrer B., Efroni S., Cohen H.Y. Reciprocal regulation between SIRT6 and miR-122 controls liver metabolism and predicts hepatocarcinoma prognosis. Cell Rep 2016; 14(2): 234–242, https://doi.org/10.1016/j.celrep.2015.12.023.
- Chen F., Feng L., Zheng Y.L., Lu J., Fan S.H., Shan Q., Zheng G.H., Wang Y.J., Wu D.M., Li M.Q., Wang Q.Q., Zhang Z.F. 2, 2’, 4, 4’-tetrabromodiphenyl ether (BDE-47) induces mitochondrial dysfunction and related liver injury via eliciting miR-34a-5p-mediated mitophagy impairment. Environ Pollut 2020; 258: 113693, https://doi.org/10.1016/j.envpol.2019.113693.
- Song L., Chen T.Y., Zhao X.J., Xu Q., Jiao R.Q., Li J.M., Kong L.D. Pterostilbene prevents hepatocyte epithelial-mesenchymal transition in fructose-induced liver fibrosis through suppressing miR-34a/Sirt1/p53 and TGF-β1/Smads signalling. Br J Pharmacol 2019; 176(11): 1619–1634, https://doi.org/10.1111/bph.14573.
- Cheng Y., Mai J., Hou T., Ping J. MicroRNA-421 induces hepatic mitochondrial dysfunction in non-alcoholic fatty liver disease mice by inhibiting sirtuin 3. Biochem Biophys Res Commun 2016; 474(1): 57–63, https://doi.org/10.1016/j.bbrc.2016.04.065.
- Chan S.Y., Zhang Y.Y., Hemann C., Mahoney C.E., Zweier J.L., Loscalzo J. MicroRNA-210 controls mitochondrial metabolism during hypoxia by repressing the iron-sulfur cluster assembly proteins ISCU1/2. Cell Metab 2009; 10(4): 273–284, https://doi.org/10.1016/j.cmet.2009.08.015.
- Liu X., Zhao H., Luo C., Du D., Huang J., Ming Q., Jin F., Wang D., Huang W. Acetaminophen responsive miR-19b modulates SIRT1/Nrf2 signaling pathway in drug-induced hepatotoxicity. Toxicol Sci 2019; 170(2): 476–488, https://doi.org/10.1093/toxsci/kfz095.
- Yadav A.K., Sata T.N., Verma D., Sah A.K., Mishra A.K., Mrinalini S., Hossain M., Pant K., Venugopal S.K. Free fatty acid-induced miR-22 inhibits gluconeogenesis via SIRT-1-mediated PGC-1α expression in nonalcoholic fatty liver disease. iLIVER 2023; 2(1): 1–9, https://doi.org/10.1016/j.iliver.2023.01.002.
- Ma S., Zhao Y., Sun J., Mu P., Deng Y. miR449a/SIRT1/PGC-1α is necessary for mitochondrial biogenesis induced by T-2 toxin. Front Pharmacol 2018; 8: 954, https://doi.org/10.3389/fphar.2017.00954.
- Jamwal S., Blackburn J.K., Elsworth J.D. PPARγ/PGC1α signaling as a potential therapeutic target for mitochondrial biogenesis in neurodegenerative disorders. Pharmacol Ther 2021; 219: 107705, https://doi.org/10.1016/j.pharmthera.2020.107705.
- Zheng Y., Chen X., Lu T., Lin Z., Liu C., Yuan D., Yuan C. miR-871-5p/PGC1α regulates aging-induced lipid deposition in hepatocytes through fatty acid β-oxidation. J Gerontol A Biol Sci Med Sci 2023; 2023: glad185, https://doi.org/10.1093/gerona/glad185.
- Liang J., Liu C., Qiao A., Cui Y., Zhang H., Cui A., Zhang S., Yang Y., Xiao X., Chen Y., Fang F., Chang Y. MicroRNA-29a-c decrease fasting blood glucose levels by negatively regulating hepatic gluconeogenesis. J Hepatol 2013; 58(3): 535–542, https://doi.org/10.1016/j.jhep.2012.10.024.
- Aharoni-Simon M., Hann-Obercyger M., Pen S., Madar Z., Tirosh O. Fatty liver is associated with impaired activity of PPARγ-coactivator 1α (PGC1α) and mitochondrial biogenesis in mice. Lab Invest 2011; 91(7): 1018–1028, https://doi.org/10.1038/labinvest.2011.55.
- Murphy M.P., Hartley R.C. Mitochondria as a therapeutic target for common pathologies. Nat Rev Drug Discov 2018; 17(12): 865–886, https://doi.org/10.1038/nrd.2018.174.
- Jeon T.I., Park J.W., Ahn J., Jung C.H., Ha T.Y. Fisetin protects against hepatosteatosis in mice by inhibiting miR-378. Mol Nutr Food Res 2013; 57(11): 1931–1937, https://doi.org/10.1002/mnfr.201300071.
- Zhang T., Zhao X., Steer C.J., Yan G., Song G. A negative feedback loop between microRNA-378 and Nrf1 promotes the development of hepatosteatosis in mice treated with a high fat diet. Metabolism 2018; 85: 183–191, https://doi.org/10.1016/j.metabol.2018.03.023.
- Guo W., Qiu Z., Wang Z., Wang Q., Tan N., Chen T., Chen Z., Huang S., Gu J., Li J., Yao M., Zhao Y., He X. MiR-199a-5p is negatively associated with malignancies and regulates glycolysis and lactate production by targeting hexokinase 2 in liver cancer. Hepatology 2015; 62(4): 1132–1144, https://doi.org/10.1002/hep.27929.
- Xu F., Yan J.J., Gan Y., Chang Y., Wang H.L., He X.X., Zhao Q. miR-885-5p negatively regulates Warburg effect by silencing hexokinase 2 in liver cancer. Mol Ther Nucleic Acids 2019; 18: 308–319, https://doi.org/10.1016/j.omtn.2019.09.002.
- Jiang J.X., Gao S., Pan Y.Z., Yu C., Sun C.Y. Overexpression of microRNA-125b sensitizes human hepatocellular carcinoma cells to 5-fluorouracil through inhibition of glycolysis by targeting hexokinase II. Mol Med Rep 2014; 10(2): 995–1002, https://doi.org/10.3892/mmr.2014.2271.
- Gray L.R., Tompkins S.C., Taylor E.B. Regulation of pyruvate metabolism and human disease. Cell Mol Life Sci 2014; 71(14): 2577–2604, https://doi.org/10.1007/s00018-013-1539-2.
- Kaplon J., Zheng L., Meissl K., Chaneton B., Selivanov V.A., Mackay G., van der Burg S.H., Verdegaal E.M., Cascante M., Shlomi T., Gottlieb E., Peeper D.S. A key role for mitochondrial gatekeeper pyruvate dehydrogenase in oncogene-induced senescence. Nature 2013; 498(7452): 109–112, https://doi.org/10.1038/nature12154.
- Zhao Y., Tran M., Wang L., Shin D., Wu J. PDK4-deficiency reprograms intrahepatic glucose and lipid metabolism to facilitate liver regeneration in mice. Hepatol Commun 2020; 4(4): 504–517, https://doi.org/10.1002/hep4.1484.
- Rodimova S.A., Kuznetsova D.S., Bobrov N.V., Gulin A.A., Reunov D.G., Karabut M.M., Shcheslavskiy V.I., Vdovina N.V., Zagainov V.E., Zagaynova E.V. Interrogation of the liver during regeneration by fluorescence lifetime imaging and mass spectrometry. IEEE J Select Topics Quantum Electron 2021; 27(4): 1–11, https://doi.org/10.1109/jstqe.2021.3053336.
- Si T., Ning X., Zhao H., Zhang M., Huang P., Hu Z., Yang L., Lin L. microRNA-9-5p regulates the mitochondrial function of hepatocellular carcinoma cells through suppressing PDK4. Cancer Gene Ther 2021; 28(6): 706–718, https://doi.org/10.1038/s41417-020-00253-w.
- Han H., Li W., Shen H., Zhang J., Zhu Y., Li Y. microRNA-129-5p, a c-Myc negative target, affects hepatocellular carcinoma progression by blocking the Warburg effect. J Mol Cell Biol 2016; 8(5): 400–410, https://doi.org/10.1093/jmcb/mjw010.
- Lin X., Qin Y., Jia J., Lin T., Lin X., Chen L., Zeng H., Han Y., Wu L., Huang S., Wang M., Huang S., Xie R., Liang L., Liu Y., Liu R., Zhang T., Li J., Wang S., Sun P., Huang W., Yao K., Xu K., Du T., Xiao D. MiR-155 enhances insulin sensitivity by coordinated regulation of multiple genes in mice. PLoS Genet 2016; 12(10): e1006308, https://doi.org/10.1371/journal.pgen.1006308.
- Cichoż-Lach H. Oxidative stress as a crucial factor in liver diseases. World J Gastroenterol 2014; 20(25): 8082, https://doi.org/10.3748/wjg.v20.i25.8082.
- Hayes J.D., Dinkova-Kostova A.T. The Nrf2 regulatory network provides an interface between redox and intermediary metabolism. Trends Biochem Sci 2014; 39(4): 199–218, https://doi.org/10.1016/j.tibs.2014.02.002.
- Teimouri M., Hosseini H., Shabani M., Koushki M., Noorbakhsh F., Meshkani R. Inhibiting miR-27a and miR-142-5p attenuate nonalcoholic fatty liver disease by regulating Nrf2 signaling pathway. IUBMB Life 2020; 72(3): 361–372, https://doi.org/10.1002/iub.2221.
- Yang J.J., Tao H., Hu W., Liu L.P., Shi K.H., Deng Z.Y., Li J. MicroRNA-200a controls Nrf2 activation by target Keap1 in hepatic stellate cell proliferation and fibrosis. Cell Signal 2014; 26(11): 2381–2389, https://doi.org/10.1016/j.cellsig.2014.07.016.
- Tao Y.C., Wang Y.H., Wang M.L., Jiang W., Wu D.B., Chen E.Q., Tang H. Upregulation of microRNA-125b-5p alleviates acute liver failure by regulating the Keap1/Nrf2/HO-1 pathway. Front Immunol 2022; 13: 988668, https://doi.org/10.3389/fimmu.2022.988668.
- Klieser E., Mayr C., Kiesslich T., Wissniowski T., Fazio P.D., Neureiter D., Ocker M. The crosstalk of miRNA and oxidative stress in the liver: from physiology to pathology and clinical implications. Int J Mol Sci 2019; 20(21): 5266, https://doi.org/10.3390/ijms20215266.
- Lu S.C., Mato J.M., Espinosa-Diez C., Lamas S. MicroRNA-mediated regulation of glutathione and methionine metabolism and its relevance for liver disease. Free Radic Biol Med 2016; 100: 66–72, https://doi.org/10.1016/j.freeradbiomed.2016.03.021.
- Zhang J., Guo J., Yang N., Huang Y., Hu T., Rao C. Endoplasmic reticulum stress-mediated cell death in liver injury. Cell Death Dis 2022; 13(12): 1051, https://doi.org/10.1038/s41419-022-05444-x.
- Pu S., Pan Y., Zhang Q., You T., Yue T., Zhang Y., Wang M. Endoplasmic reticulum stress and mitochondrial stress in drug-induced liver injury. Molecules 2023; 28(7): 3160, https://doi.org/10.3390/molecules28073160.
- Tak J., Kim S.G. Effects of toxicants on endoplasmic reticulum stress and hepatic cell fate determination. Toxicol Res 2023, https://doi.org/10.1007/s43188-023-00201-4.
- Díaz-Bulnes P., Saiz M.L., López-Larrea C., Rodríguez R.M. Crosstalk between hypoxia and ER stress response: a key regulator of macrophage polarization. Front Immunol 2020; 10: 2951, https://doi.org/10.3389/fimmu.2019.02951.
- Victor P., Sarada D., Ramkumar K.M. Crosstalk between endoplasmic reticulum stress and oxidative stress: focus on protein disulfide isomerase and endoplasmic reticulum oxidase 1. Eur J Pharmacol 2021; 892: 173749, https://doi.org/10.1016/j.ejphar.2020.173749.
- Ashraf N.U., Sheikh T.A. Endoplasmic reticulum stress and oxidative stress in the pathogenesis of non-alcoholic fatty liver disease. Free Radic Res 2015; 49(12): 1405–1418, https://doi.org/10.3109/10715762.2015.1078461.
- Kim A., Koo J.H., Lee J.M., Joo M.S., Kim T.H., Kim H., Jun D.W., Kim S.G. NRF2-mediated SIRT3 induction protects hepatocytes from ER stress-induced liver injury. FASEB J 2022; 36(3): e22170, https://doi.org/10.1096/fj.202101470r.
- Bhattarai K.R., Chaudhary M., Kim H.R., Chae H.J. Endoplasmic reticulum (ER) stress response failure in diseases. Trends Cell Biol 2020; 30(9): 672–675, https://doi.org/10.1016/j.tcb.2020.05.004.
- Wang J.M., Qiu Y., Yang Z., Kim H., Qian Q., Sun Q., Zhang C., Yin L., Fang D., Back S.H., Kaufman R.J., Yang L., Zhang K. IRE1α prevents hepatic steatosis by processing and promoting the degradation of select microRNAs. Sci Signal 2018; 11(530): eaao4617, https://doi.org/10.1126/scisignal.aao4617.
- Upton J.P., Wang L., Han D., Wang E.S., Huskey N.E., Lim L., Truitt M., McManus M.T., Ruggero D., Goga A., Papa F.R., Oakes S.A. IRE1α cleaves select microRNAs during ER stress to derepress translation of proapoptotic Caspase-2. Science 2012; 338(6108): 818–822, https://doi.org/10.1126/science.1226191.
- Yang P., Shen W., Reece E.A., Yang P. 679: the newly determined role of miR17 and its target, Txnip, in the diabetes-induced congenital malformations. Am J Obstet Gynecol 2020; 222(1): S430–S431, https://doi.org/10.1016/j.ajog.2019.11.693.
- Hochreuter M.Y., Dall M., Treebak J.T., Barrès R. MicroRNAs in non-alcoholic fatty liver disease: progress and perspectives. Mol Metab 2022; 65: 101581, https://doi.org/10.1016/j.molmet.2022.101581.
- Chitnis N.S., Pytel D., Bobrovnikova-Marjon E., Pant D., Zheng H., Maas N.L., Frederick B., Kushner J.A., Chodosh L.A., Koumenis C., Fuchs S.Y., Diehl J.A. miR-211 is a prosurvival microRNA that regulates chop expression in a PERK-dependent manner. Mol Cell 2012; 48(3): 353–364, https://doi.org/10.1016/j.molcel.2012.08.025.
- Bu Y., Yoshida A., Chitnis N., Altman B.J., Tameire F., Oran A., Gennaro V., Armeson K.E., McMahon S.B., Wertheim G.B., Dang C.V., Ruggero D., Koumenis C., Fuchs S.Y., Diehl J.A. A PERK–miR-211 axis suppresses circadian regulators and protein synthesis to promote cancer cell survival. Nat Cell Biol 2018; 20(1): 104–115, https://doi.org/10.1038/s41556-017-0006-y.
- Siwecka N., Rozpędek-Kamińska W., Wawrzynkiewicz A., Pytel D., Diehl J.A., Majsterek I. The structure, activation and signaling of IRE1 and its role in determining cell fate. Biomedicines 2021; 9(2): 156, https://doi.org/10.3390/biomedicines9020156.
- Jiang J., Qu Y., Liu X., Tang C., Wei P. Antagonistic crosstalk fine-tunes sensitivities of IRE1 and PERK signaling during unfolded protein response. BioRxiv 2020, https://doi.org/10.1101/2020.08.09.243527.
- Byrd A.E., Aragon I.V., Brewer J.W. MicroRNA-30c-2* limits expression of proadaptive factor XBP1 in the unfolded protein response. J Cell Biol 2012; 196(6): 689–698, https://doi.org/10.1083/jcb.201201077.
- Xu H., Tian Y., Tang D., Zou S., Liu G., Song J., Zhang G., Du X., Huang W., He B., Lin W., Jin L., Huang W., Yang J., Fu X. An endoplasmic reticulum stress–microRNA-26a feedback circuit in NAFLD. Hepatology 2021; 73(4): 1327–1345, https://doi.org/10.1002/hep.31428.
- Chen Z., Liu Y., Yang L., Liu P., Zhang Y., Wang X. MiR-149 attenuates endoplasmic reticulum stress-induced inflammation and apoptosis in nonalcoholic fatty liver disease by negatively targeting ATF6 pathway. Immunol Lett 2020; 222: 40–48, https://doi.org/10.1016/j.imlet.2020.03.003.
- De Sousa K.P., Rossi I., Abdullahi M., Ramirez M.I., Stratton D., Inal J.M. Isolation and characterization of extracellular vesicles and future directions in diagnosis and therapy. Wiley Interdiscip Rev Nanomed Nanobiotechnol 2023; 15(1): e1835, https://doi.org/10.1002/wnan.1835.
- Dixson A.C., Dawson T.R., Di Vizio D., Weaver A.M. Context-specific regulation of extracellular vesicle biogenesis and cargo selection. Nat Rev Mol Cell Biol 2023; 24(7): 454–476, https://doi.org/10.1038/s41580-023-00576-0.
- Jeppesen D.K., Zhang Q., Franklin J.L., Coffey R.J. Extracellular vesicles and nanoparticles: emerging complexities. Trends Cell Biol 2023; 33(8): 667–681, https://doi.org/10.1016/j.tcb.2023.01.002.
- Makarova J., Turchinovich A., Shkurnikov M., Tonevitsky A. Extracellular miRNAs and cell–cell communication: problems and prospects. Trends Biochem Sci 2021; 46(8): 640–651, https://doi.org/10.1016/j.tibs.2021.01.007.
- Hwang S., Yang Y.M. Exosomal microRNAs as diagnostic and therapeutic biomarkers in non-malignant liver diseases. Arch Pharm Res 2021; 44(6): 574–587, https://doi.org/10.1007/s12272-021-01338-2.
- Babuta M., Szabo G. Extracellular vesicles in inflammation: focus on the microRNA cargo of EVs in modulation of liver diseases. J Leukoc Biol 2021; 111(1): 75–92, https://doi.org/10.1002/jlb.3mir0321-156r.
- Mansoori B., Baradaran B., Nazari A., Gaballu F.A., Cho W.C.S., Mansoori B. MicroRNAs in the cancer cell-to-cell communication: an insight into biological vehicles. Biomed Pharmacother 2022; 153: 113449, https://doi.org/10.1016/j.biopha.2022.113449.
- Valadi H., Ekström K., Bossios A., Sjöstrand M., Lee J.J., Lötvall J.O. Exosome-mediated transfer of mRNAs and microRNAs is a novel mechanism of genetic exchange between cells. Nat Cell Biol 2007; 9(6): 654–659, https://doi.org/10.1038/ncb1596.
- Kalra H., Simpson R.J., Ji H., Aikawa E., Altevogt P., Askenase P., Bond V.C., Borràs F.E., Breakefield X., Budnik V., Buzas E., Camussi G., Clayton A., Cocucci E., Falcon-Perez J.M., Gabrielsson S., Gho Y.S., Gupta D., Harsha H.C., Hendrix A., Hill A.F., Inal J.M., Jenster G., Krämer-Albers E.M., Lim S.K., Llorente A., Lötvall J., Marcilla A., Mincheva-Nilsson L., Nazarenko I., Nieuwland R., Nolte-’t Hoen E.N., Pandey A., Patel T., Piper M.G., Pluchino S., Prasad T.S., Rajendran L., Raposo G., Record M., Reid G.E., Sánchez-Madrid F., Schiffelers R.M., Siljander P., Stensballe A., Stoorvogel W., Taylor D., Thery C., Valadi H., van Balkom B.W., Vázquez J., Vidal M., Wauben M.H., Yáñez-Mó M., Zoeller M., Mathivanan S. Vesiclepedia: a compendium for extracellular vesicles with continuous community annotation. PLoS Biol 2012; 10(12): e1001450, https://doi.org/10.1371/journal.pbio.1001450.
- Mathivanan S., Simpson R.J. ExoCarta: a compendium of exosomal proteins and RNA. Proteomics 2009; 9(21): 4997–5000, https://doi.org/10.1002/pmic.200900351.
- Murillo O.D., Thistlethwaite W., Rozowsky J., Subramanian S.L., Lucero R., Shah N., Jackson A.R., Srinivasan S., Chung A., Laurent C.D., Kitchen R.R., Galeev T., Warrell J., Diao J.A., Welsh J.A., Hanspers K., Riutta A., Burgstaller-Muehlbacher S., Shah R.V., Yeri A., Jenkins L.M., Ahsen M.E., Cordon-Cardo C., Dogra N., Gifford S.M., Smith J.T., Stolovitzky G., Tewari A.K., Wunsch B.H., Yadav K.K., Danielson K.M., Filant J., Moeller C., Nejad P., Paul A., Simonson B., Wong D.K., Zhang X., Balaj L., Gandhi R., Sood A.K., Alexander R.P., Wang L., Wu C., Wong D.T.W., Galas D.J., Van Keuren-Jensen K., Patel T., Jones J.C., Das S., Cheung K.H., Pico A.R., Su A.I., Raffai R.L., Laurent L.C., Roth M.E., Gerstein M.B., Milosavljevic A. exRNA atlas analysis reveals distinct extracellular RNA cargo types and their carriers present across human biofluids. Cell 2019; 177(2): 463–477.e15, https://doi.org/10.1016/j.cell.2019.02.018.
- Lai H., Li Y., Zhang H., Hu J., Liao J., Su Y., Li Q., Chen B., Li C., Wang Z., Li Y., Wang J., Meng Z., Huang Z., Huang S. exoRBase 2.0: an atlas of mRNA, lncRNA and circRNA in extracellular vesicles from human biofluids. Nucleic Acids Res 2022; 50(D1): D118–D128, https://doi.org/10.1093/nar/gkab1085.
- Garcia-Martin R., Wang G., Brandão B.B., Zanotto T.M., Shah S., Kumar Patel S., Schilling B., Kahn C.R. MicroRNA sequence codes for small extracellular vesicle release and cellular retention. Nature 2022; 601(7893): 446–451, https://doi.org/10.1038/s41586-021-04234-3.
- Santangelo L., Giurato G., Cicchini C., Montaldo C., Mancone C., Tarallo R., Battistelli C., Alonzi T., Weisz A., Tripodi M. The RNA-binding protein SYNCRIP is a component of the hepatocyte exosomal machinery controlling microRNA sorting. Cell Rep 2016; 17(3): 799–808, https://doi.org/10.1016/j.celrep.2016.09.031.
- Villarroya-Beltri C., Gutiérrez-Vázquez C., Sánchez-Cabo F., Pérez-Hernández D., Vázquez J., Martin-Cofreces N., Martinez-Herrera D.J., Pascual-Montano A., Mittelbrunn M., Sánchez-Madrid F. Sumoylated hnRNPA2B1 controls the sorting of miRNAs into exosomes through binding to specific motifs. Nat Commun 2013; 4(1): 2980, https://doi.org/10.1038/ncomms3980.
- Roderburg C., Mollnow T., Bongaerts B., Elfimova N., Vargas Cardenas D., Berger K., Zimmermann H., Koch A., Vucur M., Luedde M., Hellerbrand C., Odenthal M., Trautwein C., Tacke F., Luedde T. Micro-RNA profiling in human serum reveals compartment-specific roles of miR-571 and miR-652 in liver cirrhosis. PLoS One 2012; 7(3): e32999, https://doi.org/10.1371/journal.pone.0032999.
- Jopling C. Liver-specific microRNA-122: biogenesis and function. RNA Biol 2012; 9(2): 137–142, https://doi.org/10.4161/rna.18827.
- Sendi H., Mead I., Wan M., Mehrab-Mohseni M., Koch K., Atala A., Bonkovsky H.L., Bishop C.E. miR-122 inhibition in a human liver organoid model leads to liver inflammation, necrosis, steatofibrosis and dysregulated insulin signaling. PLoS One 2018; 13(7): e0200847, https://doi.org/10.1371/journal.pone.0200847.
- Hu Y., Peng X., Du G., Zhang Z., Zhai Y., Xiong X., Luo X. MicroRNA-122-5p inhibition improves inflammation and oxidative stress damage in dietary-induced non-alcoholic fatty liver disease through targeting FOXO3. Front Physiol 2022; 13: 803445, https://doi.org/10.3389/fphys.2022.803445.
- Yamada H., Ohashi K., Suzuki K., Munetsuna E., Ando Y., Yamazaki M., Ishikawa H., Ichino N., Teradaira R., Hashimoto S. Longitudinal study of circulating miR-122 in a rat model of non-alcoholic fatty liver disease. Clin Chim Acta 2015; 446: 267–271, https://doi.org/10.1016/j.cca.2015.05.002.
- O’Grady T., Njock M.S., Lion M., Bruyr J., Mariavelle E., Galvan B., Boeckx A., Struman I., Dequiedt F. Sorting and packaging of RNA into extracellular vesicles shape intracellular transcript levels. BMC Biol 2022; 20(1): 72, https://doi.org/10.1186/s12915-022-01277-4.
- Botti V., Marrone S., Cannistraro S., Bizzarri A.R. Interaction between miR4749 and human serum albumin as revealed by fluorescence, FRET, atomic force spectroscopy and computational modelling. Int J Mol Sci 2022; 23(3): 1291, https://doi.org/10.3390/ijms23031291.
- Jeppesen D.K., Fenix A.M., Franklin J.L., Higginbotham J.N., Zhang Q., Zimmerman L.J., Liebler D.C., Ping J., Liu Q., Evans R., Fissell W.H., Patton J.G., Rome L.H., Burnette D.T., Coffey R.J. Reassessment of exosome composition. Cell 2019; 177(2): 428–445.e18, https://doi.org/10.1016/j.cell.2019.02.029.
- Karimi N., Cvjetkovic A., Jang S.C., Crescitelli R., Hosseinpour Feizi M.A., Nieuwland R., Lötvall J., Lässer C. Detailed analysis of the plasma extracellular vesicle proteome after separation from lipoproteins. Cell Mol Life Sci 2018; 75(15): 2873–2886, https://doi.org/10.1007/s00018-018-2773-4.
- Sódar B.W., Kittel Á., Pálóczi K., Vukman K.V., Osteikoetxea X., Szabó-Taylor K., Németh A., Sperlágh B., Baranyai T., Giricz Z., Wiener Z., Turiák L., Drahos L., Pállinger É., Vékey K., Ferdinandy P., Falus A., Buzás E.I. Low-density lipoprotein mimics blood plasma-derived exosomes and microvesicles during isolation and detection. Sci Rep 2016; 6(1): 24316, https://doi.org/10.1038/srep24316.
- Das S.; Extracellular RNA Communication Consortium; Ansel K.M., Bitzer M., Breakefield X.O., Charest A., Galas D.J., Gerstein M.B., Gupta M., Milosavljevic A., McManus M.T., Patel T., Raffai R.L., Rozowsky J., Roth M.E., Saugstad J.A., Van Keuren-Jensen K., Weaver A.M., Laurent L.C. The extracellular RNA communication consortium: establishing foundational knowledge and technologies for extracellular RNA research. Cell 2019; 177(2): 231–242, https://doi.org/10.1016/j.cell.2019.03.023.
- Lee H., Kim S.I., Shin D., Yoon Y., Choi T.H., Cheon G.J., Kim M. Hepatic siRNA delivery using recombinant human apolipoprotein A-I in mice. Biochem Biophys Res Commun 2009; 378(2): 192–196, https://doi.org/10.1016/j.bbrc.2008.11.029.
- Niculescu L.S., Simionescu N., Sanda G.M., Carnuta M.G., Stancu C.S., Popescu A.C., Popescu M.R., Vlad A., Dimulescu D.R., Simionescu M., Sima A.V. MiR-486 and miR-92a identified in circulating HDL discriminate between stable and vulnerable coronary PLoS One 2015; 10(10): e0140958, https://doi.org/10.1371/journal.pone.0140958.
- Wagner J., Riwanto M., Besler C., Knau A., Fichtlscherer S., Röxe T., Zeiher A.M., Landmesser U., Dimmeler S. Characterization of levels and cellular transfer of circulating lipoprotein-bound microRNAs. Arterioscler Thromb Vasc Biol 2013; 33(6): 1392–1400, https://doi.org/10.1161/atvbaha.112.300741.
- Vickers K.C., Palmisano B.T., Shoucri B.M., Shamburek R.D., Remaley A.T. MicroRNAs are transported in plasma and delivered to recipient cells by high-density lipoproteins. Nat Cell Biol 2011; 13(4): 423–433, https://doi.org/10.1038/ncb2210.
- Li X., Zou X. An overview of RNA virus-encoded microRNAs. ExRNA 2019; 1: 37, https://doi.org/10.1186/s41544-019-0037-6.
- Pfeffer S., Zavolan M., Grässer F.A., Chien M., Russo J.J., Ju J., John B., Enright A.J., Marks D., Sander C., Tuschl T. Identification of virus-encoded microRNAs. Science 2004; 304(5671): 734–736, https://doi.org/10.1126/science.1096781.
- Thakur A., Kumar M. Integration of human and viral miRNAs in Epstein–Barr virus-associated tumors and implications for drug repurposing. OMICS 2023; 27(3): 93–108, https://doi.org/10.1089/omi.2023.0005.
- Wei-Ting W., Yun Y., Yang Z., Yi-Ning L., Yu-Lin W., Yi-Yi L., Yan-Jie T. EBV-microRNAs as potential biomarkers in EBV-related fever: a narrative review. Curr Mol Med 2022; 23, https://doi.org/10.2174/1566524023666221118122005.
- Pawlica P., Yario T.A., White S., Wang J., Moss W.N., Hui P., Vinetz J.M., Steitz J.A. SARS-CoV-2 expresses a microRNA-like small RNA able to selectively repress host genes. Proc Natl Acad Sci U S A 2021; 118(52): e2116668118, https://doi.org/10.1073/pnas.2116668118.
- Singh M., Chazal M., Quarato P., Bourdon L., Malabat C., Vallet T., Vignuzzi M., van der Werf S., Behillil S., Donati F., Sauvonnet N., Nigro G., Bourgine M., Jouvenet N., Cecere G. A virus-derived microRNA targets immune response genes during SARS-CoV-2 infection. EMBO Rep 2022; 23(2): e54341, https://doi.org/10.15252/embr.202154341.
- Trépo C., Chan H.L.Y., Lok A. Hepatitis B virus infection. Lancet 2014; 384(9959): 2053–2063, https://doi.org/10.1016/S0140-6736(14)60220-8.
- Yang X., Li H., Sun H., Fan H., Hu Y., Liu M., Li X., Tang H. Hepatitis B virus-encoded microRNA controls viral replication. J Virol 2017; 91(10): e01919–16, https://doi.org/10.1128/jvi.01919-16.
- Zhao X., Sun L., Mu T., Yi J., Ma C., Xie H., Liu M., Tang H. An HBV-encoded miRNA activates innate immunity to restrict HBV replication. J Mol Cell Biol 2020; 12(4): 263–276, https://doi.org/10.1093/jmcb/mjz104.
- Tang J., Xiao X., Jiang Y., Tian Y., Peng Z., Yang M., Xu Z., Gong G. miR-3 encoded by hepatitis B virus downregulates PTEN protein expression and promotes cell proliferation. J Hepatocell Carcinoma 2020; 7: 257–269, https://doi.org/10.2147/jhc.s271091.
- Loukachov V., van Dort K.A., Jansen L., Reesink H.W., Kootstra N.A. Identification of a Novel HBV encoded miRNA using next generation sequencing. Viruses 2022; 14(6): 1223, https://doi.org/10.3390/v14061223.
- Nabih H.K. The significance of HCV viral load in the incidence of HCC: a correlation between miR-122 and CCL2. J Gastrointest Canc 2020; 51(2): 412–417, https://doi.org/10.1007/s12029-019-00281-2.
- Kunden R.D., Khan J.Q., Ghezelbash S., Wilson J.A. The role of the liver-specific microRNA, miRNA-122 in the HCV replication cycle. Int J Mol Sci 2020; 21(16): 5677, https://doi.org/10.3390/ijms21165677.
- Chahal J., Gebert L.F.R., Camargo C., MacRae I.J., Sagan S.M. miR-122–based therapies select for three distinct resistance mechanisms based on alterations in RNA structure. Proc Natl Acad Sci U S A 2021; 118(33): e2103671118, https://doi.org/10.1073/pnas.2103671118.
- Panigrahi M., Palmer M.A., Wilson J.A. MicroRNA-122 regulation of hcv infections: insights from studies of miR-122-independent replication. Pathogens 2022; 11(9): 1005, https://doi.org/10.3390/pathogens11091005.
- Kim T., Croce C.M. MicroRNA: trends in clinical trials of cancer diagnosis and therapy strategies. Exp Mol Med 2023; 55(7): 1314–1321, https://doi.org/10.1038/s12276-023-01050-9.
- Zhao X., Xue X., Cui Z., Kwame Amevor F., Wan Y., Fu K., Wang C., Peng C., Li Y. microRNAs-based diagnostic and therapeutic applications in liver fibrosis. Wiley Interdiscip Rev RNA 2023; 14(4): e1773, https://doi.org/10.1002/wrna.1773.
- Ratnasari N., Lestari P., Renovaldi D., Raditya Ningsih J., Qoriansas N., Wardana T., Hakim S., Signa Aini Gumilas N., Indrarti F., Triwikatmani C., Bayupurnama P., Setyo Heriyanto D., Astuti I., Mubarika Harjana S. Potential plasma biomarkers: miRNA-29c, miRNA-21, and miRNA-155 in clinical progression of hepatocellular carcinoma patients. PLoS One 2022; 17(2): e0263298, https://doi.org/10.1371/journal.pone.0263298.
- Khare S., Khare T., Ramanathan R., Ibdah J.A. Hepatocellular carcinoma: the role of microRNAs. Biomolecules 2022; 12(5): 645, https://doi.org/10.3390/biom12050645.
- Tadokoro T., Morishita A., Masaki T. Diagnosis and therapeutic management of liver fibrosis by microRNA. Int J Mol Sci 2021; 22(15): 8139, https://doi.org/10.3390/ijms22158139.
- Kim T.H., Lee Y., Lee Y.S., Gim J.A., Ko E., Yim S.Y., Jung Y.K., Kang S., Kim M.Y., Kim H., Kim B.H., Kim J.H., Seo Y.S., Yim H.J., Yeon J.E., Um S.H., Byun K.S. Circulating miRNA is a useful diagnostic biomarker for nonalcoholic steatohepatitis in nonalcoholic fatty liver disease. Sci Rep 2021; 11(1): 14639, https://doi.org/10.1038/s41598-021-94115-6.
- Wang W., Li W., Cao L., Wang B., Liu C., Qin Y., Guo B., Huang C. Serum extracellular vesicle MicroRNAs as candidate biomarkers for acute rejection in patients subjected to liver transplant. Front Genet 2022; 13: 1015049, https://doi.org/10.3389/fgene.2022.1015049.
- de Ronde M.W.J., Ruijter J.M., Moerland P.D., Creemers E.E., Pinto-Sietsma S.J. Study design and qPCR data analysis guidelines for reliable circulating miRNA biomarker experiments: a review. Clin Chem 2018; 64(9): 1308–1318, https://doi.org/10.1373/clinchem.2017.285288.
- de Planell-Saguer M., Rodicio M.C. Analytical aspects of microRNA in diagnostics: a review. Anal Chim Acta 2011; 699(2): 134–152, https://doi.org/10.1016/j.aca.2011.05.025.
- Vanhaverbeke M., Attard R., Bartekova M., Ben-Aicha S., Brandenburger T., de Gonzalo-Calvo D., Emanueli C., Farrugia R., Grillari J., Hackl M., Kalocayova B., Martelli F., Scholz M., Wettinger S.B., Devaux Y. Peripheral blood RNA biomarkers for cardiovascular disease from bench to bedside: a position paper from the EU-CardioRNA COST action CA17129. Cardiovasc Res 2022; 118(16): 3183–3197, https://doi.org/10.1093/cvr/cvab327.
- Fan R., Xiao C., Wan X., Cha W., Miao Y., Zhou Y., Qin C., Cui T., Su F., Shan X. Small molecules with big roles in microRNA chemical biology and microRNA-targeted therapeutics. RNA Biol 2019; 16(6): 707–718, https://doi.org/10.1080/15476286.2019.1593094.
- Doghish A.S., Elballal M.S., Elazazy O., Elesawy A.E., Elrebehy M.A., Shahin R.K., Midan H.M., Sallam A.M. The role of miRNAs in liver diseases: potential therapeutic and clinical applications. Pathol Res Pract 2023; 243: 154375, https://doi.org/10.1016/j.prp.2023.154375.
- Zhu Y., Tan J.K., Wong S.K., Goon J.A. Therapeutic effects of microRNAs on nonalcoholic fatty liver disease (NAFLD) and nonalcoholic steatohepatitis (NASH): a systematic review and meta-analysis. Int J Mol Sci 2023; 24(11): 9168, https://doi.org/10.3390/ijms24119168.
- Beavers K.R., Nelson C.E., Duvall C.L. MiRNA inhibition in tissue engineering and regenerative medicine. Adv Drug Deliv Rev 2015; 88: 123–137, https://doi.org/10.1016/j.addr.2014.12.006.
- Tay F.C., Lim J.K., Zhu H., Hin L.C., Wang S. Using artificial microRNA sponges to achieve microRNA loss-of-function in cancer cells. Adv Drug Deliv Rev 2015; 81: 117–127, https://doi.org/10.1016/j.addr.2014.05.010.
- Li D., Zhang J., Li J. Role of miRNA sponges in hepatocellular carcinoma. Clin Chim Acta 2020; 500: 10–19, https://doi.org/10.1016/j.cca.2019.09.013.
- Gubu A., Su W., Zhao X., Zhang X., Fan X., Wang J., Wang Q., Tang X. Circular antisense oligonucleotides for specific RNase-H-mediated microRNA inhibition with reduced off-target effects and nonspecific immunostimulation. J Med Chem 2021; 64(21): 16046–16055, https://doi.org/10.1021/acs.jmedchem.1c01421.
- Wang Z. The principles of MiRNA-masking antisense oligonucleotides technology. Methods Mol Biol 2011; 676: 43–49, https://doi.org/10.1007/978-1-60761-863-8_3.
- Wang Z. Sponge miR-mask technology. In: MicroRNA interference technologies. Springer Berlin Heidelberg; 2009; p. 167–173, https://doi.org/10.1007/978-3-642-00489-6_11.
- Yoshioka K., Kunieda T., Asami Y., Guo H., Miyata H., Yoshida-Tanaka K., Sujino Y., Piao W., Kuwahara H., Nishina K., Hara R.I., Nagata T., Wada T., Obika S., Yokota T. Highly efficient silencing of microRNA by heteroduplex oligonucleotides. Nucleic Acids Res 2019; 47(14): 7321–7332, https://doi.org/10.1093/nar/gkz492.
- Bernardo B.C., Charchar F.J., Lin R.C., McMullen J.R. A microRNA guide for clinicians and basic scientists: background and experimental techniques. Heart Lung Circ 2012; 21(3): 131–142, https://doi.org/10.1016/j.hlc.2011.11.002.
- Ottosen S., Parsley T.B., Yang L., Zeh K., van Doorn L.J., van der Veer E., Raney A.K., Hodges M.R., Patick A.K. In vitro antiviral activity and preclinical and clinical resistance profile of miravirsen, a novel anti-hepatitis C virus therapeutic targeting the human factor miR-122. Antimicrob Agents Chemother 2015; 59(1): 599–608, https://doi.org/10.1128/aac.04220-14.
- van den Berg F.T., Rossi J.J., Arbuthnot P., Weinberg M.S. Design of effective primary microRNA mimics with different basal stem conformations. Mol Ther Nucleic Acids 2016; 5(1): e278, https://doi.org/10.1038/mtna.2015.53.
- Dickins R.A., Hemann M.T., Zilfou J.T., Simpson D.R., Ibarra I., Hannon G.J., Lowe S.W. Probing tumor phenotypes using stable and regulated synthetic microRNA precursors. Nat Genet 2005; 37(11): 1289–1295, https://doi.org/10.1038/ng1651.
- Wittrup A., Lieberman J. Knocking down disease: a progress report on siRNA therapeutics. Nat Rev Genet 2015; 16(9): 543–552, https://doi.org/10.1038/nrg3978.
- Kaushal A. Innate immune regulations and various siRNA modalities. Drug Deliv Transl Res 2023; 2023: 1–15, https://doi.org/10.1007/s13346-023-01361-4.
- Ruman U., Fakurazi S., Masarudin M.J., Hussein M.Z. Nanocarrier-based therapeutics and theranostics drug delivery systems for next generation of liver cancer nanodrug modalities. Int J Nanomedicine 2020; 15: 1437–1456, https://doi.org/10.2147/ijn.s236927.
- Tang Y., Wang X., Li J., Nie Y., Liao G., Yu Y., Li C. Overcoming the reticuloendothelial system barrier to drug delivery with a “don’t-eat-us” strategy. ACS Nano 2019; 13(11): 13015–13026, https://doi.org/10.1021/acsnano.9b05679.
- Paunovska K., Loughrey D., Dahlman J.E. Drug delivery systems for RNA therapeutics. Nat Rev Genet 2022; 23(5): 265–280, https://doi.org/10.1038/s41576-021-00439-4.
- Böttger R., Pauli G., Chao P.H., Al Fayez N., Hohenwarter L., Li S.D. Lipid-based nanoparticle technologies for liver targeting. Adv Drug Deliv Rev 2020; 154–155: 79–101, https://doi.org/10.1016/j.addr.2020.06.017.
- Yang N. An overview of viral and nonviral delivery systems for microRNA. Int J Pharma Investig 2015; 5(4): 179, https://doi.org/10.4103/2230-973x.167646.
- Hudgin R.L., Pricer W.E. Jr., Ashwell G., Stockert R.J., Morell A.G. The isolation and properties of a rabbit liver binding protein specific for asialoglycoproteins. J Biol Chem 1974; 249(17): 5536–5543, https://doi.org/10.1016/s0021-9258(20)79761-9.
- Willoughby J.L.S., Chan A., Sehgal A., Butler J.S., Nair J.K., Racie T., Shulga-Morskaya S., Nguyen T., Qian K., Yucius K., Charisse K., van Berkel T.J.C., Manoharan M., Rajeev K.G., Maier M.A., Jadhav V., Zimmermann T.S. Evaluation of GalNAc-siRNA conjugate activity in pre-clinical animal models with reduced asialoglycoprotein receptor expression. Mol Ther 2018; 26(1): 105–114, https://doi.org/10.1016/j.ymthe.2017.08.019.
- Huang Y. Preclinical and clinical advances of GalNAc-decorated nucleic acid therapeutics. Mol Ther Nucleic Acids 2017; 6: 116–132, https://doi.org/10.1016/j.omtn.2016.12.003.
- Tran S., DeGiovanni P.J., Piel B., Rai P. Cancer nanomedicine: a review of recent success in drug delivery. Clin Transl Med 2017; 6(1): e44, https://doi.org/10.1186/s40169-017-0175-0.
- El Moukhtari S.H., Garbayo E., Amundarain A., Pascual-Gil S., Carrasco-León A., Prosper F., Agirre X., Blanco-Prieto M.J. Lipid nanoparticles for siRNA delivery in cancer treatment. J Control Release 2023; 361: 130–146, https://doi.org/10.1016/j.jconrel.2023.07.054.
- Sakashita M., Mochizuki S., Sakurai K. Hepatocyte-targeting gene delivery using a lipoplex composed of galactose-modified aromatic lipid synthesized with click chemistry. Bioorg Med Chem 2014; 22(19): 5212–5219, https://doi.org/10.1016/j.bmc.2014.08.012.
- Avital Y.Y., Grønbech-Jensen N., Farago O. The thermodynamics of endosomal escape and DNA release from lipoplexes. Phys Chem Chem Phys 2016; 18(4): 2591–2596, https://doi.org/10.1039/c5cp05778g.
- Dasgupta I., Chatterjee A. Recent advances in miRNA delivery systems. Methods Protoc 2021; 4(1): 10, https://doi.org/10.3390/mps4010010.
- Silva B.F.B., Majzoub R.N., Chan C.L., Li Y., Olsson U., Safinya C.R. PEGylated cationic liposome–DNA complexation in brine is pathway-dependent. Biochim Biophys Acta 2014; 1838(1 Pt B): 398–412, https://doi.org/10.1016/j.bbamem.2013.09.008.
- Hattori Y., Tamaki K., Sakasai S., Ozaki K., Onishi H. Effects of PEG anchors in PEGylated siRNA lipoplexes on in vitro gene‑silencing effects and siRNA biodistribution in mice. Mol Med Rep 2020; 22(5): 4183–4196, https://doi.org/10.3892/mmr.2020.11525.
- Xu F., Liao J.Z., Xiang G.Y., Zhao P.X., Ye F., Zhao Q., He X.X. MiR-101 and doxorubicin codelivered by liposomes suppressing malignant properties of hepatocellular carcinoma. Cancer Med 2017; 6(3): 651–661, https://doi.org/10.1002/cam4.1016.
- Liu Y., Liu J., Quimbo A., Xia F., Yao J., Clamme J.P., Zabludoff S., Zhang J., Ying W. Anti-HSP47 siRNA lipid nanoparticle ND-L02-s0201 reverses interstitial pulmonary fibrosis in preclinical rat models. ERJ Open Res 2021; 7(2): 00733–02020, https://doi.org/10.1183/23120541.00733-2020.
- Jäger M., Schubert S., Ochrimenko S., Fischer D., Schubert U.S. Branched and linear poly(ethylene imine)-based conjugates: synthetic modification, characterization, and application. Chem Soc Rev 2012; 41(13): 4755, https://doi.org/10.1039/c2cs35146c.
- Li X., Wang B., Zhou S., Chen W., Chen H., Liang S., Zheng L., Yu H., Chu R., Wang M., Chai Z., Feng W. Surface chemistry governs the sub-organ transfer, clearance and toxicity of functional gold nanoparticles in the liver and kidney. J Nanobiotechnology 2020; 18(1): 45, https://doi.org/10.1186/s12951-020-00599-1.
- Gao S., Tian H., Guo Y., Li Y., Guo Z., Zhu X., Chen X. miRNA oligonucleotide and sponge for miRNA-21 inhibition mediated by PEI-PLL in breast cancer therapy. Acta Biomater 2015; 25: 184–193, https://doi.org/10.1016/j.actbio.2015.07.020.
- Cheng C.J., Saltzman W.M. Polymer nanoparticle-mediated delivery of microRNA inhibition and alternative splicing. Mol Pharm 2012; 9(5): 1481–1488, https://doi.org/10.1021/mp300081s.
- Park J.K., Utsumi T., Seo Y.E., Deng Y., Satoh A., Saltzman W.M., Iwakiri Y. Cellular distribution of injected PLGA-nanoparticles in the liver. Nanomedicine 2016; 12(5): 1365–1374, https://doi.org/10.1016/j.nano.2016.01.013.
- Wang F., Zhang B., Zhou L., Shi Y., Li Z., Xia Y., Tian J. Imaging dendrimer-grafted graphene oxide mediated anti-miR-21 delivery with an activatable luciferase reporter. ACS Appl Mater Interfaces 2016; 8(14): 9014–9021, https://doi.org/10.1021/acsami.6b02662.
- Reebye V., Sætrom P., Mintz P.J., Huang K.W., Swiderski P., Peng L., Liu C., Liu X., Lindkaer-Jensen S., Zacharoulis D., Kostomitsopoulos N., Kasahara N., Nicholls J.P., Jiao L.R., Pai M., Spalding D.R., Mizandari M., Chikovani T., Emara M.M., Haoudi A., Tomalia D.A., Rossi J.J., Habib N.A. Novel RNA oligonucleotide improves liver function and inhibits liver carcinogenesis in vivo. Hepatology 2014; 59(1): 216–227, https://doi.org/10.1002/hep.26669.
- Salzano G., Costa D.F., Sarisozen C., Luther E., Mattheolabakis G., Dhargalkar P.P., Torchilin V.P. Mixed nanosized polymeric micelles as promoter of doxorubicin and miRNA-34a co-delivery triggered by dual stimuli in tumor tissue. Small 2016; 12(35): 4837–4848, https://doi.org/10.1002/smll.201600925.
- Bitar A., Ahmad N.M., Fessi H., Elaissari A. Silica-based nanoparticles for biomedical applications. Drug Discov Today 2012; 17(19–20): 1147–1154, https://doi.org/10.1016/j.drudis.2012.06.014.
- Mamaeva V., Sahlgren C., Lindén M. Mesoporous silica nanoparticles in medicine — recent advances. Adv Drug Deliv Rev 2013; 65(5): 689–702, https://doi.org/10.1016/j.addr.2012.07.018.
- Sun S., Wang Y., Zhou R., Deng Z., Han Y., Han X., Tao W., Yang Z., Shi C., Hong D., Li J., Shi D., Zhang Z. Targeting and regulating of an oncogene via nanovector delivery of microRNA using patient-derived xenografts. Theranostics 2017; 7(3): 677–693, https://doi.org/10.7150/thno.16357.
- Sharma A., Cornejo C., Mihalic J., Geyh A., Bordelon D.E., Korangath P., Westphal F., Gruettner C., Ivkov R. Physical characterization and in vivo organ distribution of coated iron oxide nanoparticles. Sci Rep 2018; 8(1): 4916, https://doi.org/10.1038/s41598-018-23317-2.
- Thomson D.W., Bracken C.P., Goodall G.J. Experimental strategies for microRNA target identification. Nucleic Acids Res 2011; 39(16): 6845–6853, https://doi.org/10.1093/nar/gkr330.
- Gagnon K.T., Corey D.R. Guidelines for experiments using antisense oligonucleotides and double-stranded RNAs. Nucleic Acid Ther 2019; 29(3): 116–122, https://doi.org/10.1089/nat.2018.0772.
- Hedlund H., Du Rietz H., Johansson J.M., Eriksson H.C., Zedan W., Huang L., Wallin J., Wittrup A. Single-cell quantification and dose-response of cytosolic siRNA delivery. Nat Commun 2023; 14(1): 1075, https://doi.org/10.1038/s41467-023-36752-1.