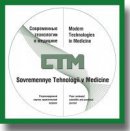
РНК-секвенирование и пространственная транскриптомика при травме спинного мозга (обзор)
Для понимания фундаментальных механизмов функционирования спинного мозга необходимо выявить полный набор клеточных типов и образуемых ими популяций, которые могут быть идентифицированы по уникальному сочетанию признаков. Технологии секвенирования РНК отдельной клетки и отдельного клеточного ядра служат эффективными инструментами для определения роли клеток разных типов в нормальных и патологических реакциях в спинном мозге. Пространственная транскриптомика сочетает эти технологии с методами получения и сохранения пространственной информации о клетках в ткани, что позволяет более точно локализовать область повреждения, детально охарактеризовать тканевые компартменты в конкретной анатомической области и анализировать патологическую картину на клеточном и молекулярном уровне.
Созданные на основе данных РНК-секвенирования отдельной клетки и отдельного клеточного ядра атласы развития технологий РНК-секвенирования и пространственной транскриптомики открывают большие возможности для формулирования новых перспективных концепций, касающихся механизмов реорганизации нейронных связей и восстановления сенсомоторных функций при травме спинного мозга. Полученные транскриптомы явились мощным ресурсом для выяснения новых функций клеток нервной ткани. С целью установления терапевтических мишеней выявляемое молекулярное разнообразие в нейронах разных типов позволяет отслеживать и сравнивать их уязвимость и регенераторный потенциал. Для выяснения причин селективной уязвимости клеток при травме спинного мозга важно располагать детальной информацией о специфике клеточных популяций у человека в сопоставлении с известными данными, полученными на экспериментальных моделях.
В предлагаемом обзоре обобщены достижения по идентификации и изучению характеристик клеток в травмированном спинном мозге на основе транскрипционного профилирования на уровне отдельной клетки или отдельного клеточного ядра.
- Côté M.P., Murray L.M., Knikou M. Spinal control of locomotion: individual neurons, their circuits and functions. Front Physiol 2018; 9: 784, https://doi.org/10.3389/fphys.2018.00784.
- Ramírez-Jarquín U.N., Tapia R. Excitatory and inhibitory neuronal circuits in the spinal cord and their role in the control of motor neuron function and degeneration. ACS Chem Neurosci 2018; 9(2): 211–216, https://doi.org/10.1021/acschemneuro.7b00503.
- Osseward P.J. II, Amin N.D., Moore J.D., Temple B.A., Barriga B.K., Bachmann L.C., Beltran F. Jr., Gullo M., Clark R.C., Driscoll S.P., Pfaff S.L., Hayashi M. Conserved genetic signatures parcellate cardinal spinal neuron classes into local and projection subsets. Science 2021; 372(6540): 385–393, https://doi.org/10.1126/science.abe0690.
- Cahoy J.D., Emery B., Kaushal A., Foo L.C., Zamanian J.L., Christopherson K.S., Xing Y., Lubischer J.L., Krieg P.A., Krupenko S.A., Thompson W.J., Barres B.A. A transcriptome database for astrocytes, neurons, and oligodendrocytes: a new resource for understanding brain development and function. J Neurosci 2008; 28(1): 264–278, https://doi.org/10.1523/jneurosci.4178-07.2008.
- Dobrott C.I., Sathyamurthy A., Levine A.J. Decoding cell type diversity within the spinal cord. Curr Opin Physiol 2019; 8: 1–6, https://doi.org/10.1016/j.cophys.2018.11.006.
- Wu H., Kirita Y., Donnelly E.L., Humphreys B.D. Advantages of single-nucleus over single-cell RNA sequencing of adult kidney: rare cell types and novel cell states revealed in fibrosis. J Am Soc Nephrol 2019; 30(1): 23–32, https://doi.org/10.1681/asn.2018090912.
- Ding J., Adiconis X., Simmons S.K., Kowalczyk M.S., Hession C.C., Marjanovic N.D., Hughes T.K., Wadsworth M.H., Burks T., Nguyen L.T., Kwon J.Y.H., Barak B., Ge W., Kedaigle A.J., Carroll S., Li S., Hacohen N., Rozenblatt-Rosen O., Shalek A.K., Villani A.C., Regev A., Levin J.Z. Systematic comparison of single-cell and single-nucleus RNA-sequencing methods. Nat Biotechnol 2020; 38(6): 737–746, https://doi.org/10.1038/s41587-020-0465-8.
- Hwang B., Lee J.H., Bang D. Single-cell RNA sequencing technologies and bioinformatics pipelines. Exp Mol Med 2018; 50(8): 1–14, https://doi.org/10.1038/s12276-018-0071-8.
- Chen G., Ning B., Shi T. Single-cell RNA-seq technologies and related computational data analysis. Front Genet 2019; 10: 317, https://doi.org/10.3389/fgene.2019.00317.
- Slovin S., Carissimo A., Panariello F., Grimaldi A., Bouché V., Gambardella G., Cacchiarelli D. Single-cell RNA sequencing analysis: a step-by-step overview. Methods Mol Biol 2021; 2284: 343–365, https://doi.org/10.1007/978-1-0716-1307-8_19.
- Cao Y., Zhu S., Yu B., Yao C. Single-cell RNA sequencing for traumatic spinal cord injury. FASEB J 2022; 36(12): e22656, https://doi.org/10.1096/fj.202200943r.
- Jovic D., Liang X., Zeng H., Lin L., Xu F., Luo Y. Single-cell RNA sequencing technologies and applications: a brief overview. Clin Transl Med 2022; 12(3): e694, https://doi.org/10.1002/ctm2.694.
- Liu Y., Liang S., Wang B., Zhao J., Zi X., Yan S., Dou T., Jia J., Wang K., Ge C. Advances in single-cell sequencing technology and its application in poultry science. Genes (Basel) 2022; 13(12): 2211, https://doi.org/10.3390/genes13122211.
- Skinnider M.A., Gautier M., Yue A., Teo Y., Kathe C., Hutson T.H., Laskaratos A., de Coucy A., Regazzi N., Aureli V., James N.D., Schneider B., Sofroniew M.V., Barraud Q., Bloch J., Anderson M.A., Squair J.W., Courtine G. The Tabulae Paralytica: multimodal single-cell and spatial atlases of spinal cord injury. bioRxiv 2023, https://doi.org/10.1101/2023.06.23.544348.
- Sathyamurthy A., Johnson K.R., Matson K.J.E., Dobrott C.I., Li L., Ryba A.R., Bergman T.B., Kelly M.C., Kelley M.W., Levine A.J. Massively parallel single nucleus transcriptional profiling defines spinal cord neurons and their activity during behavior. Cell Rep 2018; 22(8): 2216–2225, https://doi.org/10.1016/j.celrep.2018.02.003.
- Zeisel A., Hochgerner H., Lönnerberg P., Johnsson A., Memic F., van der Zwan J., Häring M., Braun E., Borm L.E., La Manno G., Codeluppi S., Furlan A., Lee K., Skene N., Harris K.D., Hjerling-Leffler J., Arenas E., Ernfors P., Marklund U., Linnarsson S. Molecular architecture of the mouse nervous system. Cell 2018; 174(4): 999–1014.e22, https://doi.org/10.1016/j.cell.2018.06.021.
- Russ D.E., Cross R.B.P., Li L., Koch S.C., Matson K.J.E., Yadav A., Alkaslasi M.R., Lee D.I., Le Pichon C.E., Menon V., Levine A.J. A harmonized atlas of mouse spinal cord cell types and their spatial organization. Nat Commun 2021; 12(1): 5722, https://doi.org/10.1038/s41467-021-25125-1.
- Li Y., Chen Y., Li X., Wu J., Pan J.Y., Cai R.X., Yang R.Y., Wang X.D. RNA sequencing screening of differentially expressed genes after spinal cord injury. Neural Regen Res 2019; 14(9): 1583–1593, https://doi.org/10.4103/1673-5374.255994.
- Zeisel A., Muñoz-Manchado A.B., Codeluppi S., Lönnerberg P., La Manno G., Juréus A., Marques S., Munguba H., He L., Betsholtz C., Rolny C., Castelo-Branco G., Hjerling-Leffler J., Linnarsson S. Brain structure. Cell types in the mouse cortex and hippocampus revealed by single-cell RNA-seq. Science 2015; 347(6226): 1138–1142, https://doi.org/10.1126/science.aaa1934.
- Masuda T., Sankowski R., Staszewski O., Böttcher C., Amann L., Sagar S., Scheiwe C., Nessler S., Kunz P., van Loo G., Coenen V.A., Reinacher P.C., Michel A., Sure U., Gold R., Grün D., Priller J., Stadelmann C., Prinz M. Spatial and temporal heterogeneity of mouse and human microglia at single-cell resolution. Nature 2019; 566(7744): 388–392, https://doi.org/10.1038/s41586-019-0924-x.
- Bomkamp C., Tripathy S.J., Bengtsson Gonzales C., Hjerling-Leffler J., Craig A.M., Pavlidis P. Transcriptomic correlates of electrophysiological and morphological diversity within and across excitatory and inhibitory neuron classes. PLoS Comput Biol 2019; 15(6): e1007113, https://doi.org/10.1371/journal.pcbi.1007113.
- Sagner A., Briscoe J. Establishing neuronal diversity in the spinal cord: a time and a Westholm J.O., La Manno G., Lundeberg J., Frisén J. Clonal relations in the mouse brain revealed by single-cell and spatial transcriptomics. Nat Neurosci 2022; 25(3): 285–294, https://doi.org/10.1038/s41593-022-01011-x.
- Stifani N. Motor neurons and the generation of spinal motor neuron diversity. Front Cell Neurosci 2014; 8: 293, https://doi.org/10.3389/fncel.2014.00293.
- Morisaki Y., Niikura M., Watanabe M., Onishi K., Tanabe S., Moriwaki Y., Okuda T., Ohara S., Murayama S., Takao M., Uchida S., Yamanaka K., Misawa H. Selective expression of Osteopontin in ALS-resistant motor neurons is a critical determinant of late phase neurodegeneration mediated by matrix metalloproteinase-9. Sci Rep 2016; 6: 27354, https://doi.org/10.1038/srep27354.
- Bączyk M., Manuel M., Roselli F., Zytnicki D. Diversity of mammalian motoneurons and motor units. Adv Neurobiol 2022; 28: 131–150, https://doi.org/10.1007/978-3-031-07167-6_6.
- Miles G.B., Hartley R., Todd A.J., Brownstone R.M. Spinal cholinergic interneurons regulate the excitability of motoneurons during locomotion. Proc Natl Acad Sci U S A 2007; 104(7): 2448–2453, https://doi.org/10.1073/pnas.0611134104.
- Bikoff J.B., Gabitto M.I., Rivard A.F., Drobac E., Machado T.A., Miri A., Brenner-Morton S., Famojure E., Diaz C., Alvarez F.J., Mentis G.Z., Jessell T.M. Spinal inhibitory interneuron diversity delineates variant motor microcircuits. Cell 2016; 165(1): 207–219, https://doi.org/10.1016/j.cell.2016.01.027.
- Bikoff J.B. Interneuron diversity and function in the spinal motor system. Curr Opin Physiol 2019; 8: 36–43, https://doi.org/10.1016/j.cophys.2018.12.013.
- Chen S., Yang G., Zhu Y., Liu Z., Wang W., Wei J., Li K., Wu J., Chen Z., Li Y., Mu S., OuYang L., Lei W. A comparative study of three interneuron types in the rat spinal cord. PLoS One 2016; 11(9): e0162969, https://doi.org/10.1371/journal.pone.0162969.
- Bertuzzi M., Ampatzis K. Spinal cholinergic interneurons differentially control motoneuron excitability and alter the locomotor network operational range. Sci Rep 2018; 8(1): 1988, https://doi.org/10.1038/s41598-018-20493-z.
- Sweeney L.B., Bikoff J.B., Gabitto M.I., Brenner-Morton S., Baek M., Yang J.H., Tabak E.G., Dasen J.S., Kintner C.R., Jessell T.M. Origin and segmental diversity of spinal inhibitory interneurons. Neuron 2018; 97(2): 341–355.e3, https://doi.org/10.1016/j.neuron.2017.12.029.
- Deska-Gauthier D., Zhang Y. The functional diversity of spinal interneurons and locomotor control. Curr Opin Physiol 2019; 8: 99–108, https://doi.org/10.1016/j.cophys.2019.01.005.
- Laliberte A.M., Goltash S., Lalonde N.R., Bui T.V. Propriospinal neurons: essential elements of locomotor control in the intact and possibly the injured spinal cord. Front Cell Neurosci 2019; 13: 512, https://doi.org/10.3389/fncel.2019.00512.
- Nascimento F., Broadhead M.J., Tetringa E., Tsape E., Zagoraiou L., Miles G.B. Synaptic mechanisms underlying modulation of locomotor-related motoneuron output by premotor cholinergic interneurons. Elife 2020; 9: e54170, https://doi.org/10.7554/elife.54170.
- Alkaslasi M.R., Piccus Z.E., Hareendran S., Silberberg H., Chen L., Zhang Y., Petros T.J., Le Pichon C.E. Single nucleus RNA-sequencing defines unexpected diversity of cholinergic neuron types in the adult mouse spinal cord. Nat Commun 2021; 12(1): 2471, https://doi.org/10.1038/s41467-021-22691-2.
- Blum J.A., Klemm S., Shadrach J.L., Guttenplan K.A., Nakayama L., Kathiria A., Hoang P.T., Gautier O., Kaltschmidt J.A., Greenleaf W.J., Gitler A.D. Single-cell transcriptomic analysis of the adult mouse spinal cord reveals molecular diversity of autonomic and skeletal motor neurons. Nat Neurosci 2021; 24(4): 572–583, https://doi.org/10.1038/s41593-020-00795-0.
- Liau E.S., Jin S., Chen Y.C., Liu W.S., Calon M., Nedelec S., Nie Q., Chen J.A. Single-cell transcriptomic analysis reveals diversity within mammalian spinal motor neurons. Nat Commun 2023; 14(1): 46, https://doi.org/10.1038/s41467-022-35574-x.
- Kaplan A., Spiller K.J., Towne C., Kanning K.C., Choe G.T., Geber A., Akay T., Aebischer P., Henderson C.E. Neuronal matrix metalloproteinase-9 is a determinant of selective neurodegeneration. Neuron 2014; 81(2): 333–348, https://doi.org/10.1016/j.neuron.2013.12.009.
- Nijssen J., Comley L.H., Hedlund E. Motor neuron vulnerability and resistance in amyotrophic lateral sclerosis. Acta Neuropathol 2017; 133(6): 863–885, https://doi.org/10.1007/s00401-017-1708-8.
- Schweingruber C., Hedlund E. The cell autonomous and non-cell autonomous aspects of neuronal vulnerability and resilience in amyotrophic lateral sclerosis. Biology (Basel) 2022; 11(8): 1191, https://doi.org/10.3390/biology11081191.
- Taylor J.P., Brown R.H. Jr., Cleveland D.W. Decoding ALS: from genes to mechanism. Nature 2016; 539(7628): 197–206, https://doi.org/10.1038/nature20413.
- Shi L.L., Zhang N., Xie X.M., Chen Y.J., Wang R., Shen L., Zhou J.S., Hu J.G., Lü H.Z. Transcriptome profile of rat genes in injured spinal cord at different stages by RNA-sequencing. BMC Genomics 2017; 18(1): 173, https://doi.org/10.1186/s12864-017-3532-x.
- Floriddia E.M., Lourenço T., Zhang S., van Bruggen D., Hilscher M.M., Kukanja P., Gonçalves Dos Santos J.P., Altınkök M., Yokota C., Llorens-Bobadilla E., Mulinyawe S.B., Grãos M., Sun L.O., Frisén J., Nilsson M., Castelo-Branco G. Distinct oligodendrocyte populations have spatial preference and different responses to spinal cord injury. Nat Commun 2020; 11(1): 5860, https://doi.org/10.1038/s41467-020-19453-x.
- Llorens-Bobadilla E., Chell J.M., Le Merre P., Wu Y., Zamboni M., Bergenstråhle J., Stenudd M., Sopova E., Lundeberg J., Shupliakov O., Carlén M., Frisén J. A latent lineage potential in resident neural stem cells enables spinal cord repair. Science 2020; 370(6512): eabb8795, https://doi.org/10.1126/science.abb8795.
- Poplawski G.H.D., Kawaguchi R., Van Niekerk E., Lu P., Mehta N., Canete P., Lie R., Dragatsis I., Meves J.M., Zheng B., Coppola G., Tuszynski M.H. Injured adult neurons regress to an embryonic transcriptional growth state. Nature 2020; 581(7806): 77–82, https://doi.org/10.1038/s41586-020-2200-5.
- Milich L.M., Choi J.S., Ryan C., Cerqueira S.R., Benavides S., Yahn S.L., Tsoulfas P., Lee J.K. Single-cell analysis of the cellular heterogeneity and interactions in the injured mouse spinal cord. J Exp Med 2021; 218(8): e20210040, https://doi.org/10.1084/jem.20210040.
- Kathe C., Skinnider M.A., Hutson T.H., Regazzi N., Gautier M., Demesmaeker R., Komi S., Ceto S., James N.D., Cho N., Baud L., Galan K., Matson K.J.E., Rowald A., Kim K., Wang R., Minassian K., Prior J.O., Asboth L., Barraud Q., Lacour S.P., Levine A.J., Wagner F., Bloch J., Squair J.W., Courtine G. The neurons that restore walking after paralysis. Nature 2022; 611(7936): 540–547, https://doi.org/10.1038/s41586-022-05385-7.
- Matson K.J.E., Russ D.E., Kathe C., Hua I., Maric D., Ding Y., Krynitsky J., Pursley R., Sathyamurthy A., Squair J.W., Levi B.P., Courtine G., Levine A.J. Single cell atlas of spinal cord injury in mice reveals a pro-regenerative signature in spinocerebellar neurons. Nat Commun 2022; 13(1): 5628, https://doi.org/10.1038/s41467-022-33184-1.
- Rodrigo Albors A., Singer G.A., Llorens-Bobadilla E., Frisén J., May A.P., Ponting C.P., Storey K.G. An ependymal cell census identifies heterogeneous and ongoing cell maturation in the adult mouse spinal cord that changes dynamically on injury. Dev Cell 2023; 58(3): 239–255.e10, https://doi.org/10.1016/j.devcel.2023.01.003.
- Yadav A., Matson K.J.E., Li L., Hua I., Petrescu J., Kang K., Alkaslasi M.R., Lee D.I., Hasan S., Galuta A., Dedek A., Ameri S., Parnell J., Alshardan M.M., Qumqumji F.A., Alhamad S.M., Wang A.P., Poulen G., Lonjon N., Vachiery-Lahaye F., Gaur P., Nalls M.A., Qi Y.A., Maric D., Ward M.E., Hildebrand M.E., Mery P.F., Bourinet E., Bauchet L., Tsai E.C., Phatnani H., Le Pichon C.E., Menon V., Levine A.J. A cellular taxonomy of the adult human spinal cord. Neuron 2023; 111(3): 328–344.e7, https://doi.org/10.1016/j.neuron.2023.01.007.
- Sun J., Qiu J., Yang Q., Ju Q., Qu R., Wang X., Wu L., Xing L. Single-cell RNA sequencing reveals dysregulation of spinal cord cell types in a severe spinal muscular atrophy mouse model. PLoS Genet 2022; 18(9): e1010392, https://doi.org/10.1371/journal.pgen.1010392.
- Chelyshev Y. More attention on segments remote from the primary spinal cord lesion site. Front Biosci (Landmark Ed) 2022; 27(8): 235, https://doi.org/10.31083/j.fbl2708235.
- Челышев Ю.А., Шаймарданова Г.Ф., Мухамедшина Я.О., Нигметзянова М.В. Глиальные барьеры при травме спинного мозга как мишень генно-клеточной терапии. Неврологический вестник 2013; 45(1): 87–93.
- Northcutt A.J., Schulz D.J. Molecular mechanisms of homeostatic plasticity in central pattern generator networks. Dev Neurobiol 2020; 80(1–2): 58–69, https://doi.org/10.1002/dneu.22727.
- Grillner S., Kozlov A. The CPGs for limbed locomotion-facts and fiction. Int J Mol Sci 2021; 22(11): 5882, https://doi.org/10.3390/ijms22115882.
- Fink K.L., Cafferty W.B. Reorganization of intact descending motor circuits to replace lost connections after injury. Neurotherapeutics 2016; 13(2): 370–381, https://doi.org/10.1007/s13311-016-0422-x.
- Wang Y., Wu W., Wu X., Sun Y., Zhang Y.P., Deng L.X., Walker M.J., Qu W., Chen C., Liu N.K., Han Q., Dai H., Shields L.B., Shields C.B., Sengelaub D.R., Jones K.J., Smith G.M., Xu X.M. Remodeling of lumbar motor circuitry remote to a thoracic spinal cord injury promotes locomotor recovery. Elife 2018; 7: e39016, https://doi.org/10.7554/elife.39016.
- Anderson M.A., Squair J.W., Gautier M., Hutson T.H., Kathe C., Barraud Q., Bloch J., Courtine G. Natural and targeted circuit reorganization after spinal cord injury. Nat Neurosci 2022; 25(12): 1584–1596, https://doi.org/10.1038/s41593-022-01196-1.
- McBride R.L., Feringa E.R. Ventral horn motoneurons 10, 20 and 52 weeks after T-9 spinal cord transection. Brain Res Bull 1992; 28(1): 57–60, https://doi.org/10.1016/0361-9230(92)90230-u.
- Yokota K., Kubota K., Kobayakawa K., Saito T., Hara M., Kijima K., Maeda T., Katoh H., Ohkawa Y., Nakashima Y., Okada S. Pathological changes of distal motor neurons after complete spinal cord injury. Mol Brain 2019; 12(1): 4, https://doi.org/10.1186/s13041-018-0422-3.
- Poplawski G.H., Tuszynski M.H. Regeneration of corticospinal axons into neural progenitor cell grafts after spinal cord injury. Neurosci Insights 2020; 15: 2633105520974000, https://doi.org/10.1177/2633105520974000.
- Jing X., Wang T., Huang S., Glorioso J.C., Albers K.M. The transcription factor Sox11 promotes nerve regeneration through activation of the regeneration-associated gene Sprr1a. Exp Neurol 2012; 233(1): 221–232, https://doi.org/10.1016/j.expneurol.2011.10.005.
- Fagoe N.D., Attwell C.L., Kouwenhoven D., Verhaagen J., Mason M.R. Overexpression of ATF3 or the combination of ATF3, c-Jun, STAT3 and Smad1 promotes regeneration of the central axon branch of sensory neurons but without synergistic effects. Hum Mol Genet 2015; 24(23): 6788–6800, https://doi.org/10.1093/hmg/ddv383.
- Nguyen M.Q., Le Pichon C.E., Ryba N. Stereotyped transcriptomic transformation of somatosensory neurons in response to injury. Elife 2019; 8: e49679, https://doi.org/10.7554/eLife.49679.
- Renthal W., Tochitsky I., Yang L., Cheng Y.C., Li E., Kawaguchi R., Geschwind D.H., Woolf C.J. Transcriptional reprogramming of distinct peripheral sensory neuron subtypes after axonal injury. Neuron 2020; 108(1): 128–144.e9, https://doi.org/10.1016/j.neuron.2020.07.026.
- Akram R., Anwar H., Javed M.S., Rasul A., Imran A., Malik S.A., Raza C., Khan I.U., Sajid F., Iman T., Sun T., Han H.S., Hussain G. Axonal regeneration: underlying molecular mechanisms and potential therapeutic targets. Biomedicines 2022; 10(12): 3186, https://doi.org/10.3390/biomedicines10123186.
- Westergard T., Rothstein J.D. Astrocyte diversity: current insights and future directions. Neurochem Res 2020; 45(6): 1298–1305, https://doi.org/10.1007/s11064-020-02959-7.
- Escartin C., Galea E., Lakatos A., O’Callaghan J.P., Petzold G.C., Serrano-Pozo A., Steinhäuser C., Volterra A., Carmignoto G., Agarwal A., Allen N.J., Araque A., Barbeito L., Barzilai A., Bergles D.E., Bonvento G., Butt A.M., Chen W.T., Cohen-Salmon M., Cunningham C., Deneen B., De Strooper B., Díaz-Castro B., Farina C., Freeman M., Gallo V., Goldman J.E., Goldman S.A., Götz M., Gutiérrez A., Haydon P.G., Heiland D.H., Hol E.M., Holt M.G., Iino M., Kastanenka K.V., Kettenmann H., Khakh B.S., Koizumi S., Lee C.J., Liddelow S.A., MacVicar B.A., Magistretti P., Messing A., Mishra A., Molofsky A.V., Murai K.K., Norris C.M., Okada S., Oliet S.H.R., Oliveira J.F., Panatier A., Parpura V., Pekna M., Pekny M., Pellerin L., Perea G., Pérez-Nievas B.G., Pfrieger F.W., Poskanzer K.E., Quintana F.J., Ransohoff R.M., Riquelme-Perez M., Robel S., Rose C.R., Rothstein J.D., Rouach N., Rowitch D.H., Semyanov A., Sirko S., Sontheimer H., Swanson R.A., Vitorica J., Wanner I.B., Wood L.B., Wu J., Zheng B., Zimmer E.R., Zorec R., Sofroniew M.V., Verkhratsky A. Reactive astrocyteplace. Development 2019; 146(22): dev182154, https://doi.org/10.1242/dev.182154.
- Ratz M., von Berlin L., Larsson L., Martin M., nomenclature, definitions, and future directions. Nat Neurosci 2021; 24(3): 312–325, https://doi.org/10.1038/s41593-020-00783-4.
- Endo F., Kasai A., Soto J.S., Yu X., Qu Z., Hashimoto H., Gradinaru V., Kawaguchi R., Khakh B.S. Molecular basis of astrocyte diversity and morphology across the CNS in health and disease. Science 2022; 378(6619): eadc9020, https://doi.org/10.1126/science.adc9020.
- Prabhakar P., Pielot R., Landgraf P., Wissing J., Bayrhammer A., van Ham M., Gundelfinger E.D., Jänsch L., Dieterich D.C., Müller A. Monitoring regional astrocyte diversity by cell type-specific proteomic labeling in vivo. Glia 2023; 71(3): 682–703, https://doi.org/10.1002/glia.24304.
- Wei H., Wu X., Withrow J., Cuevas-Diaz Duran R., Singh S., Chaboub L.S., Rakshit J., Mejia J., Rolfe A., Herrera J.J., Horner P.J., Wu J.Q. Glial progenitor heterogeneity and key regulators revealed by single-cell RNA sequencing provide insight to regeneration in spinal cord injury. Cell Rep 2023; 42(5): 112486, https://doi.org/10.1016/j.celrep.2023.112486.
- Bernal A., Arranz L. Nestin-expressing progenitor cells: function, identity and therapeutic implications. Cell Mol Life Sci 2018; 75(12): 2177–2195, https://doi.org/10.1007/s00018-018-2794-z.
- Adams K.L., Gallo V. The diversity and disparity of the glial scar. Nat Neurosci 2018; 21(1): 9–15, https://doi.org/10.1038/s41593-017-0033-9.
- Nicaise A.M., D’Angelo A., Ionescu R.B., Krzak G., Willis C.M., Pluchino S. The role of neural stem cells in regulating glial scar formation and repair. Cell Tissue Res 2022; 387(3): 399–414, https://doi.org/10.1007/s00441-021-03554-0.
- Alfaro-Cervello C., Cebrian-Silla A., Soriano-Navarro M., Garcia-Tarraga P., Matías-Guiu J., Gomez-Pinedo U., Molina Aguilar P., Alvarez-Buylla A., Luquin M.R., Garcia-Verdugo J.M. The adult macaque spinal cord central canal zone contains proliferative cells and closely resembles the human. J Comp Neurol 2014; 522(8): 1800–1817, https://doi.org/10.1002/cne.23501.
- Paniagua-Torija B., Norenberg M., Arevalo-Martin A., Carballosa-Gautam M.M., Campos-Martin Y., Molina-Holgado E., Garcia-Ovejero D. Cells in the adult human spinal cord ependymal region do not proliferate after injury. J Pathol 2018; 246(4): 415–421, https://doi.org/10.1002/path.5151.
- Dromard C., Guillon H., Rigau V., Ripoll C., Sabourin J.C., Perrin F.E., Scamps F., Bozza S., Sabatier P., Lonjon N., Duffau H., Vachiery-Lahaye F., Prieto M., Tran Van Ba C., Deleyrolle L., Boularan A., Langley K., Gaviria M., Privat A., Hugnot J.P., Bauchet L. Adult human spinal cord harbors neural precursor cells that generate neurons and glial cells in vitro. J Neurosci Res 2008; 86(9): 1916–1926, https://doi.org/10.1002/jnr.21646.
- Mothe A.J., Zahir T., Santaguida C., Cook D., Tator C.H. Neural stem/progenitor cells from the adult human spinal cord are multipotent and self-renewing and differentiate after transplantation. PLoS One 2011; 6(11): e27079, https://doi.org/10.1371/journal.pone.0027079.
- Hugnot J.P. Isolate and culture neural stem cells from the mouse adult spinal cord. Methods Mol Biol 2013; 1059: 53–63, https://doi.org/10.1007/978-1-62703-574-3_5.
- Frederico B., Martins I., Chapela D., Gasparrini F., Chakravarty P., Ackels T., Piot C., Almeida B., Carvalho J., Ciccarelli A., Peddie C.J., Rogers N., Briscoe J., Guillemot F., Schaefer A.T., Saúde L., Reis e Sousa C. DNGR-1-tracing marks an ependymal cell subset with damage-responsive neural stem cell potential. Dev Cell 2022; 57(16): 1957–1975.e9, https://doi.org/10.1016/j.devcel.2022.07.012.
- Stenudd M., Sabelström H., Llorens-Bobadilla E., Zamboni M., Blom H., Brismar H., Zhang S., Basak O., Clevers H., Göritz C., Barnabé-Heider F., Frisén J. Identification of a discrete subpopulation of spinal cord ependymal cells with neural stem cell properties. Cell Rep 2022; 38(9): 110440, https://doi.org/10.1016/j.celrep.2022.110440.
- Fiorelli R., Cebrian-Silla A., Garcia-Verdugo J.M., Raineteau O. The adult spinal cord harbors a population of GFAP-positive progenitors with limited self-renewal potential. Glia 2013; 61(12): 2100–2113, https://doi.org/10.1002/glia.22579.
- Redmond S.A., Figueres-Oñate M., Obernier K., Nascimento M.A., Parraguez J.I., López-Mascaraque L., Fuentealba L.C., Alvarez-Buylla A. Development of ependymal and postnatal neural stem cells and their origin from a common embryonic progenitor. Cell Rep 2019; 27(2): 429–441.e3, https://doi.org/10.1016/j.celrep.2019.01.088.
- Meletis K., Barnabé-Heider F., Carlén M., Evergren E., Tomilin N., Shupliakov O., Frisén J. Spinal cord injury reveals multilineage differentiation of ependymal cells. PLoS Biol 2008; 6(7): e182, https://doi.org/10.1371/journal.pbio.0060182.
- Rolls A., Shechter R., Schwartz M. The bright side of the glial scar in CNS repair. Nat Rev Neurosci 2009; 10(3): 235–241, https://doi.org/10.1038/nrn2591.
- Barnabé-Heider F., Göritz C., Sabelström H., Takebayashi H., Pfrieger F.W., Meletis K., Frisén J. Origin of new glial cells in intact and injured adult spinal cord. Cell Stem Cell 2010; 7(4): 470–482, https://doi.org/10.1016/j.stem.2010.07.014.
- Marinelli S., Basilico B., Marrone M.C., Ragozzino D. Microglia-neuron crosstalk: signaling mechanism and control of synaptic transmission. Semin Cell Dev Biol 2019; 94: 138–151, https://doi.org/10.1016/j.semcdb.2019.05.017.
- Borst K., Dumas A.A., Prinz M. Microglia: immune and non-immune functions. Immunity 2021; 54(10): 2194–2208, https://doi.org/10.1016/j.immuni.2021.09.014.
- Ball J.B., Green-Fulgham S.M., Watkins L.R. Mechanisms of microglia-mediated synapse turnover and synaptogenesis. Prog Neurobiol 2022; 218: 102336, https://doi.org/10.1016/j.pneurobio.2022.102336.
- McNamara N.B., Munro D.A.D., Bestard-Cuche N., Uyeda A., Bogie J.F.J., Hoffmann A., Holloway R.K., Molina-Gonzalez I., Askew K.E., Mitchell S., Mungall W., Dodds M., Dittmayer C., Moss J., Rose J., Szymkowiak S., Amann L., McColl B.W., Prinz M., Spires-Jones T.L., Stenzel W., Horsburgh K., Hendriks J.J.A., Pridans C., Muramatsu R., Williams A., Priller J., Miron V.E. Microglia regulate central nervous system myelin growth and integrity. Nature 2023; 613(7942): 120–129, https://doi.org/10.1038/s41586-022-05534-y.
- Li Q., Cheng Z., Zhou L., Darmanis S., Neff N.F., Okamoto J., Gulati G., Bennett M.L., Sun L.O., Clarke L.E., Marschallinger J., Yu G., Quake S.R., Wyss-Coray T., Barres B.A. Developmental heterogeneity of microglia and brain myeloid cells revealed by deep single-cell RNA sequencing. Neuron 2019; 101(2): 207–223.e10, https://doi.org/10.1016/j.neuron.2018.12.006.
- Hakim R., Zachariadis V., Sankavaram S.R., Han J., Harris R.A., Brundin L., Enge M., Svensson M. Spinal cord injury induces permanent reprogramming of microglia into a disease-associated state which contributes to functional recovery. J Neurosci 2021; 41(40): 8441–8459, https://doi.org/10.1523/jneurosci.0860-21.2021.
- Healy L.M., Zia S., Plemel J.R. Towards a definition of microglia heterogeneity. Commun Biol 2022; 5(1): 1114, https://doi.org/10.1038/s42003-022-04081-6.
- Xu L., Wang J., Ding Y., Wang L., Zhu Y.J. Current knowledge of microglia in traumatic spinal cord injury. Front Neurol 2022; 12: 796704, https://doi.org/10.3389/fneur.2021.796704.
- Hickman S., Izzy S., Sen P., Morsett L., El Khoury J. Microglia in neurodegeneration. Nat Neurosci 2018; 21(10): 1359–1369, https://doi.org/10.1038/s41593-018-0242-x.
- Zhou X., He X., Ren Y. Function of microglia and macrophages in secondary damage after spinal cord injury. Neural Regen Res 2014; 9(20): 1787–1795, https://doi.org/10.4103/1673-5374.143423.
- Kroner A., Rosas Almanza J. Role of microglia in spinal cord injury. Neurosci Lett 2019; 709: 134370, https://doi.org/10.1016/j.neulet.2019.134370.
- Ding Y., Zhang D., Wang S., Zhang X., Yang J. Hematogenous macrophages: a new therapeutic target for spinal cord injury. Front Cell Dev Biol 2021; 9: 767888, https://doi.org/10.3389/fcell.2021.767888.
- Kisucká A., Bimbová K., Bačová M., Gálik J., Lukáčová N. Activation of neuroprotective microglia and astrocytes at the lesion site and in the adjacent segments is crucial for spontaneous locomotor recovery after spinal cord injury. Cells 2021; 10(8): 1943, https://doi.org/10.3390/cells10081943.
- Kierdorf K., Masuda T., Jordão M.J.C., Prinz M. Macrophages at CNS interfaces: ontogeny and function in health and disease. Nat Rev Neurosci 2019; 20(9): 547–562, https://doi.org/10.1038/s41583-019-0201-x.
- Brennan F.H., Li Y., Wang C., Ma A., Guo Q., Li Y., Pukos N., Campbell W.A., Witcher K.G., Guan Z., Kigerl K.A., Hall J.C.E., Godbout J.P., Fischer A.J., McTigue D.M., He Z., Ma Q., Popovich P.G. Microglia coordinate cellular interactions during spinal cord repair in mice. Nat Commun 2022; 13(1): 4096, https://doi.org/10.1038/s41467-022-31797-0.
- Li C., Wu Z., Zhou L., Shao J., Hu X., Xu W., Ren Y., Zhu X., Ge W., Zhang K., Liu J., Huang R., Yu J., Luo D., Yang X., Zhu W., Zhu R., Zheng C., Sun Y.E., Cheng L. Temporal and spatial cellular and molecular pathological alterations with single-cell resolution in the adult spinal cord after injury. Signal Transduct Target Ther 2022; 7(1): 65, https://doi.org/10.1038/s41392-022-00885-4.
- Yan L., Fu J., Dong X., Chen B., Hong H., Cui Z. Identification of hub genes in the subacute spinal cord injury in rats. BMC Neurosci 2022; 23(1): 51, https://doi.org/10.1186/s12868-022-00737-5.