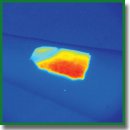
Two-Wavelength Fluorescence Monitoring and Planning of Photodynamic Therapy
The aim of the study is to develop approaches for fluorescence monitoring and planning of photodynamic therapy employing chlorine series photosensitizers.
Materials and Methods. The study included numerical simulations and experiments with optical agar phantoms of biotissue and human skin in vivo. Fluorescence imaging was used as a method of optical monitoring. Chlorine series photosensitizer Photoditazin (Veta Grand, Russia) was employed. Numerical simulation of light propagation was performed with Monte-Carlo technique for a multilayer skin model.
Results. It was demonstrated that in the case of two-wavelength fluorescence monitoring of photosensitizer penetration into the tissue the ratio of fluorescence signals excited at wavelengths of 405 and 660 nm can be used as a characteristic of photosensitizer penetration depth in biological tissue. The results of numerical simulations are in good agreement with the results of model experiments on agar phantoms and pilot in vivo experiment. Radiant exposure and absorbed light dose maps at the wavelengths of 405 and 660 nm were calculated employing Monte-Carlo technique; the dependencies of the characteristic dose values on the optical properties of the medium were analyzed.
Conclusion. Two-wave fluorescence imaging technique allows for non-invasive estimation of chlorine series photosensitizer penetration depth into the biotissue after topical application, while numerical simulation by Monte-Carlo method allows for more accurate choice of the light exposure dose for photodynamic therapy depending on optical properties of the tissue and the radiation wavelength.
- Allison R.R., Moghissi K. Photodynamic therapy (PDT): PDT mechanisms. Clin Endosc 2013; 46(1): 24–29, https://doi.org/10.5946/ce.2013.46.1.24.
- Akopov A.L., Kazakov N.V., Rusanov A.A., Karlson A. The mechanisms of photodynamic action for treating of cancer patients. Biomedical Photonics 2015; 4(2): 9–16.
- Allison R.R., Moghissi K. Oncologic photodynamic therapy: clinical strategies that modulate mechanisms of action. Photodiagnosis Photodyn Ther 2013; 10(4): 331–341, https://doi.org/10.1016/j.pdpdt.2013.03.011.
- Filonenko E.V., Serova L.G. Photodynamic therapy in clinical practice. Biomedical Photonics 2016; 5(2): 26–37.
- Juarranz A., Jaén P., Sanz-Rodríguez F., Cuevas J., González S. Photodynamic therapy of cancer. Basic principles and applications. Clin Transl Oncol 2008; 10(3): 148–154, https://doi.org/10.1007/s12094-008-0172-2.
- Kharkwal G.B., Sharma S.K., Huang Y.Y., Dai T., Hamblin M.R. Photodynamic therapy for infections: clinical applications. Lasers Surg Med 2011; 43(7): 755–767, https://doi.org/10.1002/lsm.21080.
- Biel M.A. Photodynamic therapy of head and neck cancers. Methods Mol Biol 2010; 635: 281–293, https://doi.org/10.1007/978-1-60761-697-9_18.
- Plaetzer K., Krammer B., Berlanda J., Berr F., Kiesslich T. Photophysics and photochemistry of photodynamic therapy: fundamental aspects. Lasers Med Sci 2009; 24(2): 259–268, https://doi.org/10.1007/s10103-008-0539-1.
- Allison R.R. Future PDT. Photodiagnosis Photodyn Ther 2009; 6(3–4): 231–234, https://doi.org/10.1016/j.pdpdt.2009.10.003.
- Celli J.P., Spring B.Q., Rizvi I., Evans C.L., Samkoe K.S., Verma S., Pogue B.W., Hasan T. Imaging and photodynamic therapy: mechanisms, monitoring, and optimization. Chem Rev 2010; 110(5): 2795–2838, https://doi.org/10.1021/cr900300p.
- Fei B., Wang H., Wu C., Chiu S.M. Choline PET for monitoring early tumor response to photodynamic therapy. J Nucl Med 2010; 51(1): 130–138, https://doi.org/10.2967/jnumed.109.067579.
- Wang H., Fei B. Diffusion-weighted MRI for monitoring tumor response to photodynamic therapy. J Magn Reson Imaging 2010; 32(2): 409–417, https://doi.org/10.1002/jmri.22247.
- Jo J., Lee C.H., Kopelman R., Wang X. Lifetime-resolved photoacoustic (LPA) spectroscopy for monitoring oxygen change and photodynamic therapy (PDT). Proc SPIE Int Soc Opt Eng 2016; 9708: 97081L, https://doi.org/10.1117/12.2213083.
- Gamayunov S., Turchin I., Fiks I., Korchagina K., Kleshnin M., Shakhova N. Fluorescence imaging for photodynamic therapy of non-melanoma skin malignancies — a retrospective clinical study. Photonics & Lasers in Medicine 2016; 5(2), https://doi.org/10.1515/plm-2015-0042.
- Salomatina E., Jiang B., Novak J., Yaroslavsky A.N. Optical properties of normal and cancerous human skin in the visible and near-infrared spectral range. J Biomed Opt 2006; 11(6): 064026, https://doi.org/10.1117/1.2398928.
- Tuchin V.V. Lazery i volokonnaya optika v biomeditsinskikh issledovaniyakh [Lasers and fiber optics in biomedical research]. Moscow: FIZMALIT; 2010.
- Swartling J., Bengtsson D., Terike K., Svensson J., Andersson-Engels S. Estimation of
depth of fluorescing lesions in tissue from changes in fluorescence spectra. Optical Tomography and Spectroscopy of Tissue VI 2005; 5693: 225–231, https://doi.org/10.1117/12.590145. - Swartling J., Svensson J., Bengtsson D., Terike K., Andersson-Engels S. Fluorescence spectra provide information on the depth of fluorescent lesions in tissue. Appl Opt 2005; 44(10): 1934–1941, https://doi.org/10.1364/ao.44.001934.
- Loginova D.A., Sergeeva E.A., Krainov A.D., Agrba P.D., Kirillin M.Yu. Liquid optical phantoms mimicking spectral characteristics of laboratory mouse biotissues. Quantum Electronics 2016; 46(6): 528–533, https://doi.org/10.1070/qel16133.
- Kleshnin M.S., Fiks I.I., Plekhanov V.I., Gamayunov S.V., Turchin I.V. Compact and fully automated system for monitoring photodynamic therapy, based on two LEDs and a single CCD. Laser Phys Lett 2015; 12(11): 115602, https://doi.org/10.1088/1612-2011/12/11/115602.
- Kirillin M., Perekatova V., Turchin I., Subochev P. Fluence compensation in raster-scan optoacoustic angiography. Photoacoustics 2017; 8: 59–67, https://doi.org/10.1016/j.pacs.2017.09.004.
- Loginova D.A., Sergeeva E.A., Fiks I.I., Kirillin M.Yu. Probing depth in diffuse optical spectroscopy and structured illumination imaging: a Monte Carlo study. Journal of Biomedical Photonics & Engineering 2017; 3(1): 010303, https://doi.org/10.18287/jbpe17.03.010303.
- Kirillin M.Yu., Farhat G., Sergeeva E.A., Kolios M.C., Vitkin A. Speckle statistics in OCT images: Monte Carlo simulations and experimental studies. Opt Lett 2014; 39(12): 3472–3475, https://doi.org/10.1364/ol.39.003472.
- Patwardhan S.V., Dhawan A.P., Relue P.A. Monte Carlo simulation of light-tissue interaction: three-dimensional simulation for trans-illumination-based imaging of skin lesions. IEEE Trans Biomed Eng 2005; 52(7): 1227–1236, https://doi.org/10.1109/tbme.2005.847546.
- Lopez N., Mulet R., Rodríguez R. Tumor reactive ringlet oxygen approach for Monte Carlo modeling of photodynamic therapy dosimetry. J Photochem Photobiol B 2016; 160: 383–391, https://doi.org/10.1016/j.jphotobiol.2016.04.014.