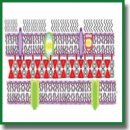
Phenotypic Plasticity as a Strategy of Bacterial Resistance and an Object of Advanced Antimicrobial Technologies (Review)
Adaptation to continuously changing habitat is one of the most important characteristics of microorganisms. A particularly effective form of adaptation is called phenotypic plasticity. This ability allows bacteria with a stable genotype to create different phenotypes in response to environmental changes. Such variability is not inheritable but is crucial for maintaining this specific bacterial population and, even more, for developing resistance to antibiotics. Studying the phenotypic plasticity, which underlies the resistance of microorganisms to traditional antibiotic therapy, is a key area of the current antimicrobial technologies. In this review, phenotypic plasticity is considered to be a strategy of bacterial survival and a mechanism for developing antibiotic resistance in dormant (resistant) cellular forms of bacteria. We suggest that studying the phenotypic variants of bacteria (L-forms; viable but nonculturable bacteria; persister cells) will result in the development of novel effective antimicrobial technologies.
- Kaufmann S.H.E. Basiswissen Immunologie. Springer Berlin Heidelberg; 2014, https://doi.org/10.1007/978-3-642-40325-5.
- Todd I., Spickett G., Fairclough L. Lecture notes: immunologe. Wiley-Blackwell; 2015.
- WHO. Antimicrobial resistance. 2015. URL: https://www.who.int.
- Errington J. L-form bacteria, cell walls and the origins of life. Open Biol 2013; 3(1): 120143–120143, https://doi.org/10.1098/rsob.120143.
- Errington J. Cell wall-deficient, L-form bacteria in the 21st century: a personal perspective. Biochem Soc Trans 2017; 45(2): 287–295, https://doi.org/10.1042/bst20160435.
- Domingue G.J. Demystifying pleomorphic forms in persistence and expression of disease: are they bacteria, and is peptidoglycan the solution? Discov Med 2010; 10(52): 234–246.
- Ferrer M., Méndez-García C., Rojo D., Barbas C., Moya A. Antibiotic use and microbiome function. Biochem Pharmacol 2017; 134: 114–126, https://doi.org/10.1016/j.bcp.2016.09.007.
- Domingue G.J., Woody H.B. Bacterial persistence and expression of disease. Clin Microbiol Rev 1997; 10(2): 320–344, https://doi.org/10.1128/cmr.10.2.320.
- Van Teeseling M.C.F., de Pedro M.A., Cava F. Determinants of bacterial morphology: from fundamentals to possibilities for antimicrobial targeting. Front Microbiol 2017; 8, https://doi.org/10.3389/fmicb.2017.01264.
- Kysela D.T., Randich A.M., Caccamo P.D., Brun Y.V. Diversity takes shape: understanding the mechanistic and adaptive basis of bacterial morphology. PLoS Biol 2016; 14(10): e1002565, https://doi.org/10.1371/journal.pbio.1002565.
- Dörr T., Davis B.M., Waldor M.K. Endopeptidase-mediated beta lactam tolerance. PLoS Pathog 2015; 11(4): e1004850, https://doi.org/10.1371/journal.ppat.1004850.
- Dziarski R., Gupta D. How innate immunity proteins kill bacteria and why they are not prone to resistance. Curr Genet 2017; 64(1): 125–129, https://doi.org/10.1007/s00294-017-0737-0.
- Sassine J., Xu M., Sidiq K.R., Emmins R., Errington J., Daniel R.A. Functional redundancy of division specific penicillin-binding proteins in Bacillus subtilis. Mol Microbiol 2017; 106(2): 304–318, https://doi.org/10.1111/mmi.13765.
- Errington J., Mickiewicz K., Kawai Y., Wu L.J. L-form bacteria, chronic diseases and the origins of life. Philos Trans R Soc Lond B Biol Sci 2016; 371(1707): 20150494, https://doi.org/10.1098/rstb.2015.0494.
- Somova L.M., Buzoleva L.S., Plekhova N.G. Ul’trastruktura patogennykh bakteriy v raznykh ekologicheskikh usloviyakh [Ultrastructure of pathogenic bacteria in different environmental conditions]. Vladivostok: Meditsina DV; 2009; 200 p.
- Kędzierska B., Hayes F. Emerging roles of toxin-antitoxin modules in bacterial pathogenesis. Molecules 2016; 21(6): 790, https://doi.org/10.3390/molecules21060790.
- Lee K.-Y., Lee B.-J. Structure, biology, and therapeutic application of toxin–antitoxin systems in pathogenic bacteria. Toxins 2016; 8(10): 305, https://doi.org/10.3390/toxins8100305.
- Hayes F. Toxins-antitoxins: plasmid maintenance, programmed cell death, and cell cycle arrest. Science 2003; 301(5639): 1496–1499, https://doi.org/10.1126/science.1088157.
- Brown B.L., Grigoriu S., Kim Y., Arruda J.M., Davenport A., Wood T.K., Peti W., Page R. Three dimensional structure of the MqsR:MqsA complex: a novel TA pair comprised of a toxin homologous to RelE and an antitoxin with unique properties. PLoS Pathog 2009; 5(12): e1000706, https://doi.org/10.1371/journal.ppat.1000706.
- Chan W.T., Balsa D., Espinosa M. One cannot rule them all: are bacterial toxins-antitoxins druggable? FEMS Microbiol Rev 2015; 39(4): 522–540, https://doi.org/10.1093/femsre/fuv002.
- Kawai Y., Mercier R., Errington J. Bacterial cell morphogenesis does not require a preexisting template structure. Curr Biol 2014; 24(8): 863–867, https://doi.org/10.1016/j.cub.2014.02.053.
- Harms A., Maisonneuve E., Gerdes K. Mechanisms of bacterial persistence during stress and antibiotic exposure. Science 2016; 354(6318): aaf4268, https://doi.org/10.1126/science.aaf4268.
- Randich A.M., Brun Y.V. Molecular mechanisms for the evolution of bacterial morphologies and growth modes. Front Microbiol 2015; 6: 580, https://doi.org/10.3389/fmicb.2015.00580.
- Ragland S.A., Criss A.K. From bacterial killing to immune modulation: recent insights into the functions of lysozyme. PLoS Pathog 2017; 13(9): e1006512, https://doi.org/10.1371/journal.ppat.1006512.
- Aguilar B., Ghaffarizadeh A., Johnson C.D., Podgorski G.J., Shmulevich I., Flann N.S. Cell death as a trigger for morphogenesis. PLoS One 2018; 13(3):e0191089, https://doi.org/10.1371/journal.pone.0191089.
- Timakov V.D., Kagan G.Ya. L-forma bakteriy i semeystvo Mycoplasmataceae v patologii [L-form bacteria and the Mycoplasmataceae family in pathology]. Moscow: Meditsina; 1973; 392 p.
- Kawai Y., Mercier R., Wu L.J., Domínguez-Cuevas P., Oshima T., Errington J. Cell growth of wall-free L-form bacteria is limited by oxidative damage. Curr Biol 2015; 25(12): 1613–1618, https://doi.org/10.1016/j.cub.2015.04.031.
- Kawai Y., Mickiewicz K., Errington J. Lysozyme counteracts β-lactam antibiotics by promoting the emergence of L-form bacteria. Cell 2018; 172(5): 1038–1049.e10, https://doi.org/10.1016/j.cell.2018.01.021.
- Fisher R.A., Gollan B., Helaine S. Persistent bacterial infections and persister cells. Nat Rev Microbiol 2017; 15(8): 453–464, https://doi.org/10.1038/nrmicro.2017.42.
- Klieneberger E. The natural occurrence of pleuropneumonia-like organism in apparent symbiosis with Streptobacillus moniliformis and other bacteria. J Pathol Bacteriol 1935; 40(1): 93–105, https://doi.org/10.1002/path.1700400108.
- Xu H.-S., Roberts N., Singleton F.L., Attwell R.W., Grimes D.J., Colwell R.R. Survival and viability of nonculturable Escherichia coli and Vibrio cholerae in the estuarine and marine environment. Microb Ecol 1982; 8(4): 313–323, https://doi.org/10.1007/bf02010671.
- Hobby G.L., Meyer K., Chaffee E. Observations on the mechanism of action of penicillin. Exp Biol Med 1942; 50(2): 281–285, https://doi.org/10.3181/00379727-50-13773.
- Casadesús J. Bacterial L-forms require peptidoglycan synthesis for cell division. BioEssays 2007; 29(12): 1189–1191, https://doi.org/10.1002/bies.20680.
- Allan E.J., Hoischen C., Gumpert J. Bacterial L-forms. Adv Appl Microbiol 2009; 1–39, https://doi.org/10.1016/s0065-2164(09)01201-5.
- McLaughlin R.W., Vali H., Lau P.C., Palfree R.G., De Ciccio A., Sirois M., Ahmad D., Villemur R., Desrosiers M., Chan E.C. Are there naturally occurring pleomorphic bacteria in the blood of healthy humans? J Clin Microbiol 2002; 40(12): 4771–4775, https://doi.org/10.1128/jcm.40.12.4771-4775.2002.
- Briers Y., Walde P., Schuppler M., Loessner M.J. How did bacterial ancestors reproduce? Lessons from L-form cells and giant lipid vesicles: multiplication similarities between lipid vesicles and L-form bacteria. BioEssays 2012; 34(12): 1078–1084, https://doi.org/10.1002/bies.201200080.
- Domínguez-Cuevas P., Mercier R., Leaver M., Kawai Y., Errington J. The rod to L-form transition of Bacillus subtilis is limited by a requirement for the protoplast to escape from the cell wall sacculus. Mol Microbiol 2011; 83(1): 52–66, https://doi.org/10.1111/j.1365-2958.2011.07920.x.
- Mercier R., Kawai Y., Errington J. General principles for the formation and proliferation of a wall-free (L-form) state in bacteria. eLife 2014; 3, https://doi.org/10.7554/elife.04629.
- Markova N., Slavchev G., Michailova L., Jourdanova M. Survival of Escherichia coli under lethal heat stress by L-form conversion. Int J Biol Sci 2010; 6(4): 303–315, https://doi.org/10.7150/ijbs.6.303.
- Markova N., Slavchev G., Michailova L. Presence of mycobacterial L-forms in human blood: challenge of BCG vaccination. Hum Vaccin Immunother 2015; 11(5): 1192–1200, https://doi.org/10.1080/21645515.2015.1016682.
- Markova N., Slavchev G., Djerov L., Nikolov A., Dimova T. Mycobacterial L-forms are found in cord blood: a potential vertical transmission of BCG from vaccinated mothers. Hum Vaccin Immunother 2016; 12(10): 2565–2571, https://doi.org/10.1080/21645515.2016.1193658.
- Markova N.D. L-form bacteria cohabitants in human blood: significance for health and diseases. Discov Med 2017; 23(128): 305–313.
- Slavchev G., Michailova L., Markova N. L-form transformation phenomenon in Mycobacterium tuberculosis associated with drug tolerance to ethambutol. Int J Mycobacteriol 2016; 5(4): 454–459, https://doi.org/10.1016/j.ijmyco.2016.06.011.
- Lovering A.L., Safadi S.S., Strynadka N.C.J. Structural perspective of peptidoglycan biosynthesis and assembly. Annu Rev Biochem 2012; 81(1): 451–478, https://doi.org/10.1146/annurev-biochem-061809-112742.
- Emami K., Guyet A., Kawai Y., Devi J., Wu L.J., Allenby N., Daniel R.A., Errington J. RodA as the missing glycosyltransferase in Bacillus subtilis and antibiotic discovery for the peptidoglycan polymerase pathway. Nat Microbiol 2017; 2(3), https://doi.org/10.1038/nmicrobiol.2016.253.
- Mercier R., Domínguez-Cuevas P., Errington J. Crucial role for membrane fluidity in proliferation of primitive cells. Cell Rep 2012; 1(5): 417–423, https://doi.org/10.1016/j.celrep.2012.03.008.
- Kashyap D.R., Rompca A., Gaballa A., Helmann J.D., Chan J., Chang C.J., Hozo I., Gupta D., Dziarski R. Peptidoglycan recognition proteins kill bacteria by inducing oxidative, thiol, and metal stress. PLoS Pathog 2014; 10(7): e1004280, https://doi.org/10.1371/journal.ppat.1004280.
- Liu C., Xu Z., Gupta D., Dziarski R. Peptidoglycan recognition proteins. J Biol Chem 2001; 276(37): 34686–34694, https://doi.org/10.1074/jbc.m105566200.
- Budin I., Szostak J.W. Expanding roles for diverse physical phenomena during the origin of life. Annu Rev Biophys 2010; 39(1): 245–263, https://doi.org/10.1146/annurev.biophys.050708.133753.
- Kawai Y., Mickiewicz K., Errington J. Lysozyme counteracts β-lactam antibiotics by promoting the emergence of L-form bacteria. Cell 2018; 172(5): 1038–1049, https://doi.org/10.1016/j.cell.2018.01.021.
- Justice S.S., Hunstad D.A., Cegelski L., Hultgren S.J. Morphological plasticity as a bacterial survival strategy. Nat Rev Microbiol 2008; 6(2): 162–168, https://doi.org/10.1038/nrmicro1820.
- Asnani P.J., Gill K. Biological properties of L-forms and their parent bacteria. Acta Microbiol Acad Sci Hung 1980; 27(2): 131–134.
- Ferguson C.M.J., Booth N.A., Allan E.J. An ELISA for the detection of Bacillus subtilis L-form bacteria confirms their symbiosis in strawberry. Lett Appl Microbiol 2000; 31(5): 390–394, https://doi.org/10.1046/j.1472-765x.2000.00834.x.
- Tedeschi G.G., Amici D., Sprovieri G., Vecchi A. Staphylococcus epidermidis in the circulating blood of normal and thrombocytopenic human subjects: immunological data. Experientia 1976; 32(12): 1600–1602, https://doi.org/10.1007/bf01924475.
- Seel W., Flegler A., Zunabovic-Pichler M., Lipski A. Increased isoprenoid quinone concentration modulates membrane fluidity in Listeria monocytogenes at low growth temperatures. J Bacteriol 2018; 200(13): e00148-18, https://doi.org/10.1128/jb.00148-18.
- Chintalapati S., Kiran M.D., Shivaji S. Role of membrane lipid fatty acids in cold adaptation. Cell Mol Biol 2004; 50(5): 631–642.
- Andryukov B.G., Somova L.M., Timchenko N.F. Fatty acid as an object of research of temperature adaptation strategies psychrophiles. Zdorov’e. Meditsinskaya ekologiya. Nauka 2015; 3(61): 43–49.
- Studer P., Staubli T., Wieser N., Wolf P., Schuppler M., Loessner M.J. Proliferation of Listeria monocytogenes L-form cells by formation of internal and external vesicles. Nat Commun 2016; 7(1), https://doi.org/10.1038/ncomms13631.
- Bigger J. Treatment of staphylococcal infections with penicillin by intermittent sterilisation. Lancet 1944; 244(6320): 497–500, https://doi.org/10.1016/s0140-6736(00)74210-3.
- Balaban N.Q. Bacterial persistence as a phenotypic switch. Science 2004; 305(5690): 1622–1625, https://doi.org/10.1126/science.1099390.
- Han J., Shi W., Xu X., Wang S., Zhang S., He L., Sun X., Zhang Y. Conditions and mutations affecting Staphylococcus aureus L-form formation. Microbiology 2015; 161(1): 57–66, https://doi.org/10.1099/mic.0.082354-0.
- Onwuamaegbu M., Belcher R., Soare C. Cell wall-deficient bacteria as a cause of infections: a review of the clinical significance. J Int Med Res 2005; 33(1): 1–20, https://doi.org/10.1177/147323000503300101.
- Beaman B.L., Scates S.M. Role of L-forms of Nocardia caviae in the development of chronic mycetomas in normal and immunodeficient murine models. Infect Immun 1981; 33(3): 893–907.
- Gupta R.S. Origin of diderm (gram-negative) bacteria: antibiotic selection pressure rather than endosymbiosis likely led to the evolution of bacterial cells with two membranes. Antonie Van Leeuwenhoek 2011; 100(2): 171–182, https://doi.org/10.1007/s10482-011-9616-8.
- Bertaux F., Marguerat S., Shahrezaei V. Division rate, cell size and proteome allocation: impact on gene expression noise and implications for the dynamics of genetic circuits. R Soc Open Sci 2018; 5(3): 172234, https://doi.org/10.1098/rsos.172234.
- Glover W.A., Yang Y., Zhang Y. Insights into the molecular basis of L-form formation and survival in Escherichia coli. PLoS One 2009; 4(10): e7316, https://doi.org/10.1371/journal.pone.0007316.
- Dhar N., McKinney J.D. Microbial phenotypic heterogeneity and antibiotic tolerance. Curr Opin Microbiol 2007; 10(1): 30–38, https://doi.org/10.1016/j.mib.2006.12.007.
- Allison K.R., Brynildsen M.P., Collins J.J. Metabolite-enabled eradication of bacterial persisters by aminoglycosides. Nature 2011; 473(7346): 216–220, https://doi.org/10.1038/nature10069.
- Barth V.C., Rodrigues B.Á., Bonatto G.D., Gallo S.W., Pagnussatti V.E., Ferreira C.A.S., de Oliveira S.D. Heterogeneous persister cells formation in Acinetobacter baumannii. PLoS One 2013; 8(12): e84361, https://doi.org/10.1371/journal.pone.0084361.
- Day T. Interpreting phenotypic antibiotic tolerance and persister cells as evolution via epigenetic inheritance. Mol Ecol 2016; 25(8): 1869–1882, https://doi.org/10.1111/mec.13603.
- Amato S.M., Brynildsen M.P. Persister heterogeneity arising from a single metabolic stress. Curr Biol 2015; 25(16): 2090–2098, https://doi.org/10.1016/j.cub.2015.06.034.
- Lewis K. Persister cells. Annu Rev Microbiol 2010; 64(1): 357–372, https://doi.org/10.1146/annurev.micro.112408.134306.
- Levin B.R., Concepción-Acevedo J., Udekwu K.I. Persistence: a copacetic and parsimonious hypothesis for the existence of non-inherited resistance to antibiotics. Curr Opin Microbiol 2014; 21: 18–21, https://doi.org/10.1016/j.mib.2014.06.016.
- Orman M.A., Brynildsen M.P. Inhibition of stationary phase respiration impairs persister formation in E. coli. Nat Commun 2015; 6(1), https://doi.org/10.1038/ncomms8983.
- Patra P., Klumpp S. Population dynamics of bacterial persistence. PLoS One 2013; 8(5): e62814, https://doi.org/10.1371/journal.pone.0062814.
- Kwan B.W., Chowdhury N., Wood T.K. Combatting bacterial infections by killing persister cells with mitomycin C. Environ Microbiol 2015; 17(11): 4406–4414, https://doi.org/10.1111/1462-2920.12873.
- Chowdhury N., Wood T.L., Martínez-Vázquez M., García-Contreras R., Wood T.K. DNA-crosslinker cisplatin eradicates bacterial persister cells. Biotechnol Bioeng 2016; 113(9): 1984–1992, https://doi.org/10.1002/bit.25963.
- Lock R.L., Harry E.J. Cell-division inhibitors: new insights for future antibiotics. Nat Rev Drug Discov 2008; 7(4): 324–38, https://doi.org/10.1038/nrd2510.
- Belov A.B., Kuzin A.A. Health care-associated sapronous infections: problematic questions of epidemiological theory. Permskiy meditsinskiy zhurnal 2017; 34(4): 94–102.
- Nemeth J., Oesch G., Kuster S.P. Bacteriostatic versus bactericidal antibiotics for patients with serious bacterial infections: systematic review and meta-analysis. J Antimicrob Chemother 2015; 70(2): 382–395, https://doi.org/10.1093/jac/dku379.
- McShan A.C., De Guzman R.N. The bacterial type III secretion system as a target for developing new antibiotics. Chem Biol Drug Des 2014; 85(1): 30–42, https://doi.org/10.1111/cbdd.12422.
- Spellberg B., Guidos R., Gilbert D., Bradley J., Boucher H.W., Scheld W.M., Bartlett J.G., Edwards J. Jr.; Infectious Diseases Society of America. The epidemic of antibiotic-resistant infections: a call to action for the medical community from the Infectious Diseases Society of America. Clin Infect Dis 2008; 46(2): 155–164, https://doi.org/10.1086/524891.
- Kint C.I., Verstraeten N., Fauvart M., Michiels J. New-found fundamentals of bacterial persistence. Trends Microbiol 2012; 20(12): 577–585, https://doi.org/10.1016/j.tim.2012.08.009.
- Lewis K. Platforms for antibiotic discovery. Nat Rev Drug Discov 2013; 12(5): 371–387, https://doi.org/10.1038/nrd3975.
- Maisonneuve E., Gerdes K. Molecular mechanisms underlying bacterial persisters. Cell 2014; 157(3): 539–548, https://doi.org/10.1016/j.cell.2014.02.050.
- Van den Bergh B., Michiels J.E., Fauvart M., Michiels J. Should we develop screens for multi-drug antibiotic tolerance? Expert Rev Anti Infect Ther 2016; 14(7): 613–616, https://doi.org/10.1080/14787210.2016.1194754.
- Lewis K., Shan Y. Persister awakening. Mol Cell 2016; 63(1): 3–4, https://doi.org/10.1016/j.molcel.2016.06.025.
- Amato S.M., Fazen C.H., Henry T.C., Mok W.W.K., Orman M.A., Sandvik E.L., Volzing K.G., Brynildsen M.P. The role of metabolism in bacterial persistence. Front Microbiol 2014; 5, https://doi.org/10.3389/fmicb.2014.00070.
- Michiels J.E., Van den Bergh B., Verstraeten N., Michiels J. Molecular mechanisms and clinical implications of bacterial persistence. Drug Resist Updat 2016; 29: 76–89, https://doi.org/10.1016/j.drup.2016.10.002.
- Wood T.K. Combatting bacterial persister cells. Biotechnol Bioeng 2015; 113(3): 476–483, https://doi.org/10.1002/bit.25721.
- Maisonneuve E., Castro-Camargo M., Gerdes K. (p)pGpp controls bacterial persistence by stochastic induction of toxin-antitoxin activity. Cell 2013; 154(5): 1140–1150, https://doi.org/10.1016/j.cell.2013.07.048.
- Manav M.C., Beljantseva J., Bojer M.S., Tenson T., Ingmer H., Hauryliuk V., Brodersen D.E. Structural basis for (p)ppGpp synthesis by the Staphylococcus aureus small alarmone synthetase RelP. J Biol Chem 2018; 293(9): 3254–3264, https://doi.org/10.1074/jbc.ra117.001374.
- Beljantseva J., Kudrin P., Jimmy S., Ehn M., Pohl R., Varik V., Tozawa Y., Shingler V., Tenson T., Rejman D., Hauryliuk V. Molecular mutagenesis of ppGpp: turning a RelA activator into an inhibitor. Sci Rep 2017; 7(1): 41839, https://doi.org/10.1038/srep41839.
- Syal K., Flentie K., Bhardwaj N., Maiti K., Jayaraman N., Stallings C.L., Chatterji D. Synthetic (p)ppGpp analogue is an inhibitor of stringent response in mycobacteria. Antimicrob Agents Chemother 2017; 61(6): e00443-17, https://doi.org/10.1128/aac.00443-17.
- Geiger T., Goerke C., Fritz M., Schäfer T., Ohlsen K., Liebeke M., Lalk M., Wolz C. Role of the (p)ppGpp synthase RSH, a RelA/SpoT homolog, in stringent response and virulence of Staphylococcus aureus. Infect Immun 2010; 78(5): 1873–1883, https://doi.org/10.1128/iai.01439-09.
- Hauryliuk V., Atkinson G.C., Murakami K.S., Tenson T., Gerdes K. Recent functional insights into the role of (p)ppGpp in bacterial physiology. Nat Rev Microbiol 2015; 13(5): 298–309, https://doi.org/10.1038/nrmicro3448.
- Ogura T., Hiraga S. Mini-F plasmid genes that couple host cell division to plasmid proliferation. Proc Natl Acad Sci USA 1983; 80(15): 4784–4788, https://doi.org/10.1073/pnas.80.15.4784.
- Page R., Peti W. Toxin-antitoxin systems in bacterial growth arrest and persistence. Nat Chem Biol 2016; 12(4): 208–214, https://doi.org/10.1038/nchembio.2044.
- Aizenman E., Engelberg-Kulka H., Glaser G. An Escherichia coli chromosomal “addiction module” regulated by guanosine [corrected] 3’,5’-bispyrophosphate: a model for programmed bacterial cell death. Proc Natl Acad Sci U S A 1996; 93(12): 6059–6063, https://doi.org/10.1073/pnas.93.12.6059.
- Christensen S.K., Maenhaut-Michel G., Mine N., Gottesman S., Gerdes K., Van Melderen L. Overproduction of the Lon protease triggers inhibition of translation in Escherichia coli: involvement of the yefM-yoeB toxin-antitoxin system. Mol Microbiol 2004; 51(6): 1705–1717, https://doi.org/10.1046/j.1365-2958.2003.03941.x.
- Van Melderen L., Saavedra De Bast M. Bacterial toxin–antitoxin systems: more than selfish entities? PLoS Genetics 2009; 5(3): e1000437, https://doi.org/10.1371/journal.pgen.1000437.
- Wen Y., Behiels E., Devreese B. Toxin-antitoxin systems: their role in persistence, biofilm formation, and pathogenicity. Pathog Dis 2014; 70(3): 240–249, https://doi.org/10.1111/2049-632x.12145.
- Van Melderen L. Toxin–antitoxin systems: why so many, what for? Curr Opin Microbiol 2010; 13(6): 781–785, https://doi.org/10.1016/j.mib.2010.10.006.
- Thakur Z., Dharra R., Saini V., Kumar A., Mehta P.K. Insights from the protein-protein interaction network analysis of Mycobacterium tuberculosis toxinantitoxin systems. Bioinformation 2017; 13(11): 380–387, https://doi.org/10.6026/97320630013380.
- Ghafourian S., Raftari M., Sadeghifard N., Sekawi Z. Toxin-antitoxin systems: classification, biological function and application in biotechnology. Curr Issues Mol Biol 2014, 16: 9–14, https://doi.org/10.21775/cimb.016.009.
- Hayes F., Kędzierska B. Regulating toxin-antitoxin expression: controlled detonation of intracellular molecular timebombs. Toxins 2014; 6(1): 337–358, https://doi.org/10.3390/toxins6010337.
- Maleki A., Ghafourian S., Pakzad I., Badakhsh B., Sadeghifard N. mazE antitoxin of toxin antitoxin system and fbpA as reliable targets to eradication of Neisseria meningitidis. Curr Pharm Des 2018; 24(11): 1204–1210, https://doi.org/10.2174/1381612824666171213094730.
- Jaén-Luchoro D., Aliaga-Lozano F., Gomila R.M., Gomila M., Salvà-Serra F., Lalucat J., Bennasar-Figueras A. First insights into a type II toxin-antitoxin system from the clinical isolate Mycobacterium sp. MHSD3, similar to epsilon/zeta systems. PLoS One 2017; 12(12): e0189459, https://doi.org/10.1371/journal.pone.0189459.
- Wood T.K. Strategies for combating persister cell and biofilm infections. Microb Biotechnol 2017; 10(5): 1054–1056, https://doi.org/10.1111/1751-7915.12774.
- Cui P., Xu T., Zhang W.H., Zhang Y. Molecular mechanisms of bacterial persistence and phenotypic antibiotic resistance. Yi Chuan 2016; 38(10): 859–871.
- Wexselblatt E., Oppenheimer-Shaanan Y., Kaspy I., London N., Schueler-Furman O., Yavin E., Glaser G., Katzhendler J., Ben-Yehuda S. Relacin, a novel antibacterial agent targeting the stringent response. PLoS Pathog 2012; 8(9): e1002925, https://doi.org/10.1371/journal.ppat.1002925.
- Kim J.S., Chowdhury N., Yamasaki R., Wood T.K. Viable but non-culturable and persistence describe the same bacterial stress state. Environ Microbiol 2018; 20(6): 2038–2048, https://doi.org/10.1111/1462-2920.14075.
- Winther K.S., Roghanian M., Gerdes K. Activation of the stringent response by loading of RelA-tRNA complexes at the ribosomal A-site. Mol Cell 2018; 70(1): 95–105.e4, https://doi.org/10.1016/j.molcel.2018.02.033.
- Somov G.P., Buzoleva L.S. Adaptatsiya patogennykh bakteriy k abioticheskim faktoram okruzhayushchey sredy [Adaptation of pathogenic bacteria to abiotic environmental factors]. Vladivostok: OAO “Poligrafkombinat”; 2004; 168 p.
- Oliver J.D. The viable but non-culturable state in the human pathogen Vibrio vulnificus. FEMS Microbiol Lett 1995; 133(3): 203–208, https://doi.org/10.1111/j.1574-6968.1995.tb07885.x.
- Oliver J.D. Recent findings on the viable but nonculturable state in pathogenic bacteria. FEMS Microbiol Rev 2010; 34(4): 415–425, https://doi.org/10.1111/j.1574-6976.2009.00200.x.
- Day A.P., Oliver J.D. Changes in membrane fatty acid composition during entry of Vibrio vulnificus into the viable but nonculturable state. J Microbiol 2004; 42(2): 69–73.
- Xiao X., Tian C., Yu Y., Wu H. Detection of viable but nonculturable Escherichia coli O157:H7 using propidium monoazide treatments and qPCR. Can J Microbiol 2013; 59(3): 157–163, https://doi.org/10.1139/cjm-2012-0577.
- Nowakowska J., Oliver J.D. Resistance to environmental stresses by Vibrio vulnificus in the viable but nonculturable state. FEMS Microbiol Ecol 2013; 84(1): 213–222, https://doi.org/10.1111/1574-6941.12052.
- Troitskaia V.V., Chetina E.V., Aliapkina I.S., Litvin V.Yu., Gintsburg A.L. Non-culturable forms of Yersinia pseudotuberculosis in the soils of a natural focus of pseudotuberculosis. Zhurnal mikrobiologii epidemiologii i immunobiologii 1996; 5: 13–15.
- Litvin V.Yu., Gintsburg A.L., Pushkareva V.I., Romanova Yu.M., Boev B.V. Epidemiologicheskie aspekty ekologii bakteriy [Epidemiological aspects of the ecology of bacteria]. Moscow: Farmus-print; 1998; 229 p.
- Barer M.R., Gribbon L.T., Harwood C.R., Nwoguh C.E. The viable but non-culturable hypothesis and medical bacteriology. Rev Med Microbiol 1993; 4(4): 183–191, https://doi.org/10.1097/00013542-199310000-00001.
- Rivers B., Steck T.R. Viable but nonculturable uropathogenic bacteria are present in the mouse urinary tract following urinary tract infection and antibiotic therapy. Urological Research 2001; 29(1): 60–66, https://doi.org/10.1007/s002400000151.
- Baffone W., Citterio B., Vittoria E., Casaroli A., Campana R., Falzano L., Donelli G. Retention of virulence in viable but non-culturable halophilic Vibrio spp. Int J Food Microbiol 2003; 89(1): 31–39, https://doi.org/10.1016/s0168-1605(03)00102-8.
- Pienaar J.A., Singh A., Barnard T.G. The viable but non-culturable state in pathogenic Escherichia coli: a general review. Afr J Lab Med 2016; 5(1): 368, https://doi.org/10.4102/ajlm.v5i1.368.
- Bamford R.A., Smith A., Metz J., Glover G., Titball R.W., Pagliara S. Investigating the physiology of viable but non-culturable bacteria by microfluidics and time-lapse microscopy. BMC Biol 2017; 15(1): 121, https://doi.org/10.1186/s12915-017-0465-4.
- Potgieter M., Bester J., Kell D.B., Pretorius E. The dormant blood microbiome in chronic, inflammatory diseases. FEMS Microbiol Rev 2015; 39(4): 567–591, https://doi.org/10.1093/femsre/fuv013.
- Del Mar Lleò M., Benedetti D., Tafi M.C., Signoretto C., Canepari P. Inhibition of the resuscitation from the viable but non-culturable state in Enterococcus faecalis. Environ Microbiol 2007; 9(9): 2313–2320, https://doi.org/10.1111/j.1462-2920.2007.01345.x.
- Ayrapetyan M., Williams T.C., Oliver J.D. Bridging the gap between viable but non-culturable and antibiotic persistent bacteria. Trends Microbiol 2015; 23(1): 7–13, https://doi.org/10.1016/j.tim.2014.09.004.
- Li L., Mendis N., Trigui H., Oliver J.D., Faucher S.P. The importance of the viable but non-culturable state in human bacterial pathogens. Front Microbiol 2014; 5: 258, https://doi.org/10.3389/fmicb.2014.00258.
- Ayrapetyan M., Williams T.C., Baxter R., Oliver J.D. Viable but nonculturable and persister cells coexist stochastically and are induced by human serum. Infect Immun 2015; 83(11): 4194–4203, https://doi.org/10.1128/iai.00404-15.
- Kim J.-S., Wood T.K. Tolerant, growing cells from nutrient shifts are not persister cells. mBio 2017; 8(2): e00354-17, https://doi.org/10.1128/mbio.00354-17.
- Ayrapetyan M., Williams T.C., Oliver J.D. Interspecific quorum sensing mediates the resuscitation of viable but nonculturable vibrios. Appl Environ Microbiol 2014; 80(8): 2478–2483, https://doi.org/10.1128/aem.00080-14.
- Zhao X., Zhong J., Wei C., Lin C.-W., Ding T. Current perspectives on viable but non-culturable state in foodborne pathogens. Front Microbiol 2017; 8: 580, https://doi.org/10.3389/fmicb.2017.00580.
- Orman M.A., Brynildsen M.P. Establishment of a method to rapidly assay bacterial persister metabolism. Antimicrob Agents Chemother 2013; 57(9): 4398–4409, https://doi.org/10.1128/aac.00372-13.
- Dietersdorfer E., Kirschner A., Schrammel B., Ohradanova-Repic A., Stockinger H., Sommer R., Walochnik J., Cervero-Aragó S. Starved viable but non-culturable (VBNC) Legionella strains can infect and replicate in amoebae and human macrophages. Water Res 2018; 141: 428–438, https://doi.org/10.1016/j.watres.2018.01.058.
- Wang X., Wood T.K. Toxin-antitoxin systems influence biofilm and persister cell formation and the general stress response. Appl Environ Microbiol 2011; 77(16): 5577–5583, https://doi.org/10.1128/aem.05068-11.
- Ramamurthy T., Ghosh A., Pazhani G.P., Shinoda S. Current perspectives on viable but non-culturable (VBNC) pathogenic bacteria. Front Public Health 2014; 2: 103, https://doi.org/10.3389/fpubh.2014.00103.
- Kuehl B., Marten S.-M., Bischoff Y., Brenner-Weiß G., Obst U. MALDI-ToF mass spectrometry-multivariate data analysis as a tool for classification of reactivation and non-culturable states of bacteria. Anal Bioanal Chem 2011; 401(5): 1593–1600, https://doi.org/10.1007/s00216-011-5227-5.
- Su X., Zhang S., Mei R., Zhang Y., Hashmi M.Z., Liu J., Lin H., Ding L., Sun F. Resuscitation of viable but non-culturable bacteria to enhance the cellulose-degrading capability of bacterial community in composting. Microb Biotechnol 2018; 11(3): 527–536, https://doi.org/10.1111/1751-7915.13256.
- Majeed M., Majeed S., Nagabhushanam K., Punnapuzha A., Philip S., Mundkur L. Rapid assessment of viable but non-culturable Bacillus coagulans MTCC 5856 in commercial formulations using Flow cytometry. PLoS One 2018; 13(2): e0192836, https://doi.org/10.1371/journal.pone.0192836.
- Liu Y., Wang C., Tyrrell G., Hrudey S.E., Li X.-F. Induction of Escherichia coli O157:H7 into the viable but non-culturable state by chloraminated water and river water, and subsequent resuscitation. Environ Microbiol Rep 2009; 1(2): 155–161, https://doi.org/10.1111/j.1758-2229.2009.00024.x.
- Pinto D., Almeida V., Almeida Santos M., Chambel L. Resuscitation of Escherichia coli VBNC cells depends on a variety of environmental or chemical stimuli. J Appl Microbiol 2011; 110(6): 1601–1611, https://doi.org/10.1111/j.1365-2672.2011.05016.x.
- Zeng B., Zhao G., Cao X., Yang Z., Wang C., Hou L. Formation and resuscitation of viable but nonculturable Salmonella typhi. Biomed Res Int 2013; 2013: 1–7, https://doi.org/10.1155/2013/907170.
- Mukamolova G.V., Murzin A.G., Salina E.G., Demina G.R., Kell D.B., Kaprelyants A.S., Young M. Muralytic activity of Micrococcus luteus Rpf and its relationship to physiological activity in promoting bacterial growth and resuscitation. Mol Microbiol 2006; 59(1): 84–98, https://doi.org/10.1111/j.1365-2958.2005.04930.x.
- Lennon J.T., Jones S.E. Microbial seed banks: the ecological and evolutionary implications of dormancy. Nat Rev Microbiol 2011; 9(2): 119–130, https://doi.org/10.1038/nrmicro2504.
- Harms A., Fino C., Sørensen M.A., Semsey S., Gerdes K. Prophages and growth dynamics confound experimental results with antibiotic-tolerant persister cells. mBio 2017; 8(6): e01964-17, https://doi.org/10.1128/mbio.01964-17.
- Nikitushkin V.D., Demina G.R., Kaprelyants A.S. Rpf proteins are the factors of reactivation of the dormant forms of actinobacteria. Biochemistry (Mosc) 2016; 81(13): 1719–1734, https://doi.org/10.1134/s0006297916130095.