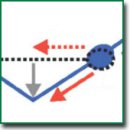
Mechanosensitivity of Cells and Its Role in the Regulation of Physiological Functions and the Implementation of Physiotherapeutic Effects (Review)
Regulatory signals in the body are not limited to chemical and electrical ones. There is another type of important signals for cells: those aremechanical signals (coming from the environment or arising from within the body), which have been less known in the literature. The review summarizes new information on the mechanosensitivity of various cells of connective tissue and nervous system. Participation of mechanical stimuli in the regulation of growth, development, differentiation, and functioning of tissues is described. The data focus on bone remodeling, wound healing, neurite growth, and the formation of neural networks. Mechanotransduction, cellular organelles, and mechanosensitive molecules involved in these processes are discussed as well as the role of the extracellular matrix. The importance of mechanical characteristics of cells in the pathogenesis of diseases is highlighted. Finally, the possible role of mechanosensitivity in mediating the physiotherapeutic effects is addressed.
- Boeri L., Albani D., Raimondi M.T., Jacchetti E. Mechanical regulation of nucleocytoplasmic translocation in mesenchymal stem cells: characterization and methods for investigation. Biophys Rev 2019; 11(5): 817–831, https://doi.org/10.1007/s12551-019-00594-3.
- Petrov K.B. The concept of myoviscerofascial connections of internal organs. Manual’naya meditsina 1994; 8: 5–11.
- Ingber D.E., Wang N., Stamenovic D. Tensegrity, cellular biophysics, and the mechanics of living systems. Rep Prog Phys 2014; 77(4): 046603, https://doi.org/10.1088/0034-4885/77/4/046603.
- Hamant O., Inoue D., Bouchez D., Dumais J., Mjolsness E. Are microtubules tension sensors? Nat Commun 2019; 10(1): 2306, https://doi.org/10.1038/s41467-019-10207-y.
- Serov V.V., Shekhter A.B. Connective tissue as a single system. Terapevticheskij arhiv 1984; 56(5): 6–12.
- Shekhter A.B., Berchenko G.N. Fibroblasts and connective tissue development: ultrastructural aspects of biosynthesis, fibrillogenesis and collagen catabolism. Arhiv patologii 1995; 3: 13.
- Jansen K.A., Donato D.M., Balcioglu H.E., Schmidt T., Danen E.H.J., Koenderink G.H. A guide to mechanobiology: where biology and physics meet. Biochim Biophys Acta 2015; 1853(11 Pt B): 3043–3052, https://doi.org/10.1016/j.bbamcr.2015.05.007.
- Franze K. The mechanical control of nervous system development. Development 2013; 140(15): 3069–3077, https://doi.org/10.1242/dev.079145.
- Uzer G., Rubin C.T., Rubin J. Cell mechanosensitivity is enabled by the LINC nuclear complex. Curr Mol Biol Rep 2016; 2(1): 36–47, https://doi.org/10.1007/s40610-016-0032-8.
- Henderson J.T., Shannon G., Veress A.I., Neu C.P. Direct measurement of intranuclear strain distributions and RNA synthesis in single cells embedded within native tissue. Biophys J 2013; 105(10): 2252–2261, https://doi.org/10.1016/j.bpj.2013.09.054.
- Wang N., Tytell J.D., Ingber D.E. Mechanotransduction at a distance: mechanically coupling the extracellular matrix with the nucleus. Nat Rev Mol Cell Biol 2009; 10: 75–82, https://doi.org/10.1038/nrm2594.
- Bishop J.E., Rhodes S., Laurent G.J., Low R.B., Stirewalt W.S. Increased collagen synthesis and decreased collagen degradation in right ventricular by pressure overload. Cardiovasc Res 1994; 28(10): 1581–1585, https://doi.org/10.1093/cvr/28.10.1581.
- Zhukova T.V., Kot Yu.G., Persky E.E. The possible mechanism of the participation of the aorta’s tensile stress in age-associated sclerosis. Vіsnik Kharkіvs’kogo natsіonal’nogo unіversitetu іm. V.N. Karazіna. Serіya: Bіologіya 2006; 729(3): 21–24.
- Zhukova T.V. Influence of mexanical stress — straine on same strucural propertiese of collagene’s molecule. Vіsnik Kharkіvs’kogo natsіonal’nogo unіversitetu іm. V.N. Karazіna. Serіya: Bіologіya 2005; 709(1–2): 39–43.
- Shekhter A.B., Nikolaev A.V., Berchenko G.N. Wound healing as an autoregulatory process and collagen stimulating mechanism. Arhiv patologii 1977; 5: 25.
- Huang X., Das R., Patel A., Nguyen T.D. Physical stimulations for bone and cartilage regeneration. Regen Eng Transl Med 2018; 4(4): 216–237, https://doi.org/10.1007/s40883-018-0064-0.
- Arpornmaeklong P., Pripatnanont P., Kittidumkerng W., Mitarnun W. Effects of autogenous growth factors on heterotopic bone formation of osteogenic cells in small animal model. J Craniomaxillofac Surg 2012; 40(4): 332–340, https://doi.org/10.1016/j.jcms.2011.05.006.
- Xin M., Yang Y., Zhang D., Wang J., Chen S., Zhou D. Attenuation of hind-limb suspension-induced bone loss by curcumin is associated with reduced oxidative stress and increased vitamin D receptor expression. Osteoporos Int 2015; 26(11): 2665–2676, https://doi.org/10.1007/s00198-015-3153-7.
- Sibonga J.D., Spector E.R., Johnston S.L., Tarver W.J. Evaluating bone loss in ISS astronauts. Aerosp Med Hum Perform 2015; 86(12 Suppl): A38–A44, https://doi.org/10.3357/AMHP.EC06.2015.
- Ng A.H., Omelon S., Variola F., Allo B., Willett T.L., Alman B.A., Grynpas M.D. Adynamic bone decreases bone toughness during aging by affecting mineral and matrix. J Bone Miner Res 2016; 31(2): 369–379, https://doi.org/10.1002/jbmr.2702.
- Meyers V.E., Zayzafoon M., Douglas J.T., McDonald J.M. RhoA and cytoskeletal disruption mediate reduced osteoblastogenesis and enhanced adipogenesis of human mesenchymal stem cells in modeled microgravity. J Bone Miner Res 2005; 20(10): 1858–1866, https://doi.org/10.1359/JBMR.050611.
- Moerman E.J., Teng K., Lipschitz D.A., Lecka-Czernik B. Aging activates adipogenic and suppresses osteogenic programs in mesenchymal marrow stroma/stem cells: the role of PPAR-γ2 transcription factor and TGF-β/BMP signaling pathways. Aging Cell 2004; 3(6): 379–389, https://doi.org/10.1111/j.1474-9728.2004.00127.x.
- Ozcivici E., Luu Y.K., Adler B., Qin Y.X., Rubin J., Judex S., Rubin C.T. Mechanical signals as anabolic agents in bone. Nat Rev Rheumatol 2010; 6(1): 50–59, https://doi.org/10.1038/nrrheum.2009.239.
- Moon M.Y., Kim H.J., Choi B.Y., Sohn M., Chung T.N., Suh S.W. Zinc promotes adipose-derived mesenchymal stem cell proliferation and differentiation towards a neuronal fate. Stem Cells Int 2018; 2018: 5736535, https://doi.org/10.1155/2018/5736535.
- Le W., Yao J. The effect of myostatin (GDF-8) on proliferation and tenocyte differentiation of rat bone marrow-derived mesenchymal stem cells. J Hand Surg Asian Pac 2017; 22(2): 200–207, https://doi.org/10.1142/S0218810417500253.
- Sun M., Chi G., Li P., Lv S., Xu J., Xu Z., Xia Y., Tan Y., Xu J., Li L., Li Y. Effects of matrix stiffness on the morphology, adhesion, proliferation and osteogenic differentiation of mesenchymal stem cells. Int J Med Sci 2018; 15(3): 257–268, https://doi.org/10.7150/ijms.21620.
- Chen Y.J., Huang C.H., Lee I.C., Lee Y.T., Chen M.H., Young T.H. Effects of cyclic mechanical stretching on the mRNA expression of tendon/ligament-related and osteoblast-specific genes in human mesenchymal stem cells. Connect Tissue Res 2008; 49(1): 7–14, https://doi.org/10.1080/03008200701818561.
- Huang Y., Jia X., Bai K., Gong X., Fan Y. Effect of fluid shear stress on cardiomyogenic differentiation of rat bone marrow mesenchymal stem cells. Arch Med Res 2010; 41(7): 497–505, https://doi.org/10.1016/j.arcmed.2010.10.002.
- Huang C.Y., Hagar K.L., Frost L.E., Sun Y., Cheung H.S. Effects of cyclic compressive loading on chondrogenesis of rabbit bone-marrow derived mesenchymal stem cells. Stem Cells 2004; 22(3): 313–323, https://doi.org/10.1634/stemcells.22-3-313.
- Sittichokechaiwut A., Edwards J.H., Scutt A.M., Reilly G.C. Short bouts of mechanical loading are as effective as dexamethasone at inducing matrix production by human bone marrow mesenchymal stem cell. Eur Cell Mater 2010; 20: 45–57, https://doi.org/10.22203/eCM.v020a05.
- Sen B., Xie Z., Case N., Styner M., Rubin C.T., Rubin J. Mechanical signal influence on mesenchymal stem cell fate is enhanced by incorporation of refractory periods into the loading regimen. J Biomech 2011; 44(4): 593–599, https://doi.org/10.1016/j.jbiomech.2010.11.022.
- Eming S.A., Martin P., Tomic-Canic M. Wound repair and regeneration: mechanisms, signaling, and translation. Sci Transl Med 2014: 6(265): 265sr6, https://doi.org/10.1126/scitranslmed.3009337.
- Schneider D., Wickstrom S.A. Force generation and transmission in keloid fibroblasts: dissecting the role of mechanosensitive molecules in cell function. Exp Dermatol 2015; 24(8): 574–575, https://doi.org/10.1111/exd.12753.
- Harn H.I., Wang Y.K., Hsu C.K., Ho Y.T., Huang Y.W., Chiu W.T., Lin H.H., Cheng C.M., Tang M.J. Mechanical coupling of cytoskeletal elasticity and force generation is crucial for understanding the migrating nature of keloid fibroblasts. Exp Dermatol 2015; 4(8): 579–584, https://doi.org/10.1111/exd.12731.
- Franze K., Janmey P.A., Guck J. Mechanics in neuronal development and repair. Annu Rev Biomed Eng 2013; 15: 227–251, https://doi.org/10.1146/annurev-bioeng-071811-150045.
- Siechen S., Yang S., Chiba A., Saif T. Mechanical tension contributes to clustering of neurotransmitter vesicles at presynaptic terminals. Proc Natl Acad Sci U S A 2009; 106(31): 12611–12616, https://doi.org/10.1073/pnas.0901867106.
- Suter D.M., Miller K.E. The emerging role of forces in axonal elongation. Prog Neurobiol 2011; 94(2): 91–101, https://doi.org/10.1016/j.pneurobio.2011.04.002.
- Mayer M., Depken M., Bois J.S., Jülicher F., Grill S.W. Anisotropies in cortical tension reveal the physical basis of polarizing cortical flows. Nature 2010; 467(7315): 617–621, https://doi.org/10.1038/nature09376.
- Christ A.F., Franze K., Gautier H., Moshayedi P., Fawcett J., Franklin R.J., Karadottir R.T., Guck J. Mechanical difference between white and gray matter in the rat cerebellum measured by scanning force microscopy. J Biomech 2010; 43(15): 2986–2992, https://doi.org/10.1016/j.jbiomech.2010.07.002.
- Sack I., Beierbach B., Wuerfel J., Klatt D., Hamhaber U., Papazoglou S., Martus P., Braun J. The impact of aging and gender on brain viscoelasticity. Neuroimage 2009; 46(3): 652–657, https://doi.org/10.1016/j.neuroimage.2009.02.040.
- Lu Y.B., Iandiev I., Hollborn M., Körber N., Ulbricht E., Hirrlinger P.G., Pannicke T., Wei E.Q., Bringmann A., Wolburg H., Wilhelmsson U., Pekny M., Wiedemann P., Reichenbach A., Käs J.A. Reactive glial cells: increased stiffness correlates with increased intermediate filament expression. FASEB J 2011; 25(2): 624–631, https://doi.org/10.1096/fj.10-163790.
- Cai L., Zhang L., Dong J., Wang S. Photocured biodegradable polymer substrates of varying stiffness and microgroove dimensions for promoting nerve cell guidance and differentiation. Langmuir 2012; 28(34): 12557–12568, https://doi.org/10.1021/la302868q.
- Jagielska A., Norman A.L., Whyte G., Vliet K.J., Guck J., Franklin R.J. Mechanical environment modulates biological properties of oligodendrocyte progenitor cells. Stem Cells Dev 2012; 21(16): 2905–2914, https://doi.org/10.1089/scd.2012.0189.
- Xu G., Bayly P.V., Taber L.A. Residual stress in the adult mouse brain. Biomech Model Mechanobiol 2009: 8(4): 253–262, https://doi.org/10.1007/s10237-008-0131-4.
- Chang Y.J., Tsai C.J., Tseng F.G., Chen T.J., Wang T.W. Micropatterned stretching system for the investigation of mechanical tension on neural stem cells behavior. Nanomedicine 2013: 9(3): 345–355, https://doi.org/10.1016/j.nano.2012.07.008.
- Norman L.L., Aranda-Espinoza H. Cortical neuron outgrowth is insensitive to substrate stiffness. Cell Mol Bioeng 2010; 3: 398–414, https://doi.org/10.1007/s12195-010-0137-8.
- Koch D., Rosoff W.J., Jiang J., Geller H.M., Urbach J.S. Strength in the periphery: growth cone biomechanics and substrate rigidity response in peripheral and central nervous system neurons. Biophys J 2012; 102(3): 452–460, https://doi.org/10.1016/j.bpj.2011.12.025.
- Xu G., Knutsen A.K., Dikranian K., Kroenke C.D., Bayly P.V., Taber L.A. Axons pull on the brain, but tension does not drive cortical folding. J Biomech Eng 2010; 132(7): 071013, https://doi.org/10.1115/1.4001683.
- Ayali A. The function of mechanical tension in neuronal and network development. Integr Biol (Camb) 2010; 2(4): 178–182, https://doi.org/10.1039/b927402b.
- Pfister B.J., Iwata A., Meaney D.F., Smith D.H. Extreme stretch growth of integrated axons. J Neurosci 2004; 24(36): 7978–7983, https://doi.org/10.1523/JNEUROSCI.1974-04.2004.
- Anava S., Greenbaum A., Ben Jacob E., Hanein Y., Ayali A. The regulative role of neurite mechanical tension in network development. Biophys J 2009; 96(4): 1661–1670, https://doi.org/10.1016/j.bpj.2008.10.058.
- Franze K., Gerdelmann J., Weick M., Betz T., Pawlizak S., Lakadamyali M., Bayer J., Rillich K., Gögler M., Lu Y.B., Reichenbach A., Janmey P., Käs J. Neurite branch retraction is caused by a threshold-dependent mechanical impact. Biophys J 2009; 97(7): 1883–1890, https://doi.org/10.1016/j.bpj.2009.07.033.
- Van Essen D.C. A tension-based theory of morphogenesis and compact wiring in the central nervous system. Nature 1997; 385(6614): 313–318, https://doi.org/10.1038/385313a0.
- Ahmed W.W., Li T.C., Rubakhin S.S., Chiba A., Sweedler J.V., Saif T.A. Mechanical tension modulates local and global vesicle dynamics in neurons. Cell Mol Bioeng 2012; 5(2): 155–164, https://doi.org/10.1007/s12195-012-0223-1.
- Ronan L., Voets N., Rua C., Alexander-Bloch A., Hough M., Mackay C., Crow T.J., James A., Giedd J.N., Fletcher P.C. Differential tangential expansion as a mechanism for cortical gyrification. Cereb Cortex 2014; 24(8): 2219–2228, https://doi.org/10.1093/cercor/bht082.
- Herculano-Houzel S., Mota B., Wong P., Kaas J.H. Connectivity-driven white matter scaling and folding in primate cerebral cortex. Proc Natl Acad Sci U S A 2010; 107(44): 19008–19013, https://doi.org/10.1073/pnas.1012590107.
- Stanton A.E., Tong X., Yang F. Extracellular matrix type modulates mechanotransduction of stem cells. Acta Biomater 2019; 96: 310–320, https://doi.org/10.1016/j.actbio.2019.06.048.
- Theocharis A.D., Skandalis S.S., Gialeli C., Karamanos N.K. Extracellular matrix structure. Adv Drug Deliv Rev 2016; 97: 4–27, https://doi.org/10.1016/j.addr.2015.11.001.
- Hassan A., Sapir L., Nitsan I., Greenblatt Ben-El R.T., Halachmi N., Salzberg A., Tzlil S. A change in ECM composition affects sensory organ mechanics and function. Cell Rep 2019; 27(8): 2272–2280, https://doi.org/10.1016/j.celrep.2019.04.092.
- Mohammed D., Versaevel M., Bruyère C., Alaimo L., Luciano M., Vercruysse E., Procès A., Gabriele S. Innovative tools for mechanobiology: unraveling outside-in and inside-out mechanotransduction. Front Bioeng Biotechnol 2019; 7: 162, https://doi.org/10.3389/fbioe.2019.00162.
- Gortazar A.R., Martin-Millan M., Bravo B., Plotkin L.I., Bellido T. Crosstalk between caveolin-1/extracellular signal-regulated kinase (ERK) and β-catenin survival pathways in osteocyte mechanotransduction. J Biol Chem 2013; 288(12): 8168–8175, https://doi.org/10.1074/jbc.M112.437921.
- Eijkelkamp N., Quick K., Wood J.N. Transient receptor potential channels and mechanosensation. Annu Rev Neurosci 2013; 36: 519–546, https://doi.org/10.1146/annurev-neuro-062012-170412.
- Uzer G., Fuchs R.K., Rubin J., Thompson W.R. Concise review: plasma and nuclear membranes convey mechanical information to regulate mesenchymal stem cell lineage. Stem Cells 2016; 34(6): 1455–1463, https://doi.org/10.1002/stem.2342.
- Zhang H., Labouesse M. Signalling through mechanical inputs: a coordinated process. J Cell Sci 2012; 125(Pt 13): 3039–3049, https://doi.org/10.1242/jcs.093666.
- Brohawn S.G., Su Z., MacKinnon R. Mechanosensitivity is mediated directly by the lipid membrane in TRAAK and TREK1 K+ channels. Proc Natl Acad Sci U S A 2014; 111(9): 3614–3619, https://doi.org/10.1073/pnas.1320768111.
- Wu J., Lewis A.H., Grandl J. Touch, tension, and transduction — the function and regulation of piezo ion channels. Trends Biochem Sci 2017; 42(1): 57–71, https://doi.org/10.1016/j.tibs.2016.09.004.
- Chubinskiy-Nadezhdin V.I., Vasileva V.Y., Pugovkina N.A., Vassilieva I.O., Morachevskaya E.A., Nikolsky N.N., Negulyaev Y.A. Local calcium signalling is mediated by mechanosensitive ion channels in mesenchymal stem cells. Biochem Biophys Res Commun 2017; 482(4): 563–568, https://doi.org/10.1016/j.bbrc.2016.11.074.
- Ogneva I.V. Cell mechanosensitivity: mechanical properties and interaction with gravitational field. Biomed Res Int 2013; 2013: 598461, https://doi.org/10.1155/2013/598461.
- Uzieliene I., Bernotas P., Mobasheri A., Bernotiene E. The role of physical stimuli on calcium channels in chondrogenic differentiation of mesenchymal stem cells. Int J Mol Sci 2018; 19(10): 2998, https://doi.org/10.3390/ijms19102998.
- Sastry S.K., Burridge K. Focal adhesions: a nexus for intracellular signaling and cytoskeletal dynamics. Exp Cell Res 2000; 261(1): 25–36, https://doi.org/10.1006/excr.2000.5043.
- Maniotis A.J., Chen C.S., Ingber D.E. Demonstration of mechanical connections between integrins cytoskeletal filaments, and nucleoplasm that stabilize nuclear structure. Proc Natl Acad Sci U S A 1997; 94(3): 849–854, https://doi.org/10.1073/pnas.94.3.849.
- Burridge K., Wittchen E.S. The tension mounts: stress fibers as force-generating mechanotransducers. J Cell Biol 2013; 200(1): 9–19, https://doi.org/10.1083/jcb.201210090.
- Ingber D.E. Tensegrity-based mechanosensing from macro to micro. Prog Biophys Mol Biol 2008; 97(2–3): 163–179, https://doi.org/10.1016/j.pbiomolbio.2008.02.005.
- Fraldi M., Palumbo S., Carotenuto A.R., Cutolo A., Deseri L., Pugno N. Buckling soft tensegrities: fickle elasticity and configurational switching in living cells. J Mech Phys Solids 2019; 124: 299–324, https://doi.org/10.1016/j.jmps.2018.10.017.
- Thorpe S.D., Lee D.A. Dynamic regulation of nuclear architecture and mechanics — a rheostatic role for the nucleus in tailoring cellular mechanosensitivity. Nucleus 2017; 8(3): 287–300, https://doi.org/10.1080/19491034.2017.1285988.
- Kang K.S., Robling A.G. New insights into Wnt-Lrp5/6-β-catenin signaling in mechanotransduction. Front Endocrinol (Lausanne) 2014; 5: 246, https://doi.org/10.3389/fendo.2014.00246.
- Niehrs C. The complex world of WNT receptor signalling. Nat Rev Mol Cell Biol 2012; 13(12): 767–779, https://doi.org/10.1038/nrm3470.
- Hadjiargyrou M., Lombardo F., Zhao S., Ahrens W., Joo J., Ahn H., Jurman M., White D.W., Rubin C.T. Transcriptional profiling of bone regeneration. Insight into the molecular complexity of wound repair. J Biol Chem 2002; 277(33): 30177–30182, https://doi.org/10.1074/jbc.M203171200.
- Chen Z., Zhang Y., Liang C., Chen L., Zhang G., Qian A. Mechanosensitive miRNAs and bone formation. Int J Mol Sci 2017; 18(8): E1684, https://doi.org/10.3390/ijms18081684.
- Park J., Wada S., Ushida T., Akimoto T. The microRNA-23a has limited roles in bone formation and homeostasis in vivo. Physiol Res 2015; 64(5): 711–719, https://doi.org/10.33549/physiolres.932901.
- Liu L., Liu M., Li R., Liu H., Du L., Chen H., Zhang Y., Zhang S., Liu D. MicroRNA-503-5p inhibits stretch-induced osteogenic differentiation and bone formation. Cell Biol Int 2017; 41(2): 112–123, https://doi.org/10.1002/cbin.10704.
- Zuo B., Zhu J., Li J., Wang C., Zhao X., Cai G., Li Z., Peng J., Wang P., Shen C., Huang Y., Xu J., Zhang X., Chen X. MicroRNA-103a functions as a mechanosensitive microRNA to inhibit bone formation through targeting Runx2. J Bone Miner Res 2015; 30: 330–345, https://doi.org/10.1002/jbmr.2352.
- Sera S.R., Zur Nieden N.I. MicroRNA regulation of skeletal development. Curr Osteoporos Rep 2017; 15(4): 353–366, https://doi.org/10.1007/s11914-017-0379-7.
- Luan X., Zhou X., Trombetta-eSilva J., Francis M., Gaharwar A.K., Atsawasuwan P., Diekwisch T.G.H. MicroRNAs and periodontal homeostasis. J Dent Res 2017; 96(5): 491–500, https://doi.org/10.1177/0022034516685711.
- Gennari L., Bianciardi S., Merlotti D. MicroRNAs in bone diseases. Osteoporos Int 2017; 28(4): 1191–1213, https://doi.org/10.1007/s00198-016-3847-5.
- Li C.J., Cheng P., Liang M.K., Chen Y.S., Lu Q., Wang J.Y., Xia Z.Y., Zhou H.D., Cao X., Xie H., Liao E.Y., Luo X.H. MicroRNA-188 regulates age-related switch between osteoblast and adipocyte differentiation. J Clin Invest 2015; 125(4): 1509–1522, https://doi.org/10.1172/JCI77716.
- Fedorchak G.R., Kaminski A., Lammerding J. Cellular mechanosensing: getting to the nucleus of it all. Prog Biophys Mol Biol 2014; 115(2–3): 76–92, https://doi.org/10.1016/j.pbiomolbio.2014.06.009.
- Navarro A.P., Collins M.A., Folker E.S. The nucleus is a conserved mechanosensation and mechanoresponse organelle. Cytoskeleton (Hoboken) 2016; 73(2): 59–67, https://doi.org/10.1002/cm.21277.
- Belaadi N., Aureille J., Guilluy C. Under pressure: mechanical stress management in the nucleus. Cells 2016; 5(2): 27, https://doi.org/10.3390/cells5020027.
- Lombardi M.L., Jaalouk D.E., Shanahan C.M., Burke B., Roux K.J., Lammerding J. The interaction between nesprins and sun proteins at the nuclear envelope is critical for force transmission between the nucleus and cytoskeleton. J Biol Chem 2011; 286: 26743–26753, https://doi.org/10.1074/jbc.M111.233700.
- Hieda M. Signal transduction across the nuclear envelope: role of the LINC complex in bidirectional signaling. Cells 2019; 8(2): 124, https://doi.org/10.3390/cells8020124.
- Ketema M., Sonnenberg A. Nesprin-3: a versatile connector between the nucleus and the cytoskeleton. Biochem Soc Trans 2011; 39(6): 1719–1724, https://doi.org/10.1042/BST20110669.
- Garcia A., Rodriguez Matas J.F., Raimondi M.T. Modeling of the mechano-chemical behaviour of the nuclear pore complex: current research and perspectives. Integr Biol (Camb) 2016; 8(10): 1011–1021, https://doi.org/10.1039/c6ib00153j.
- Donnaloja F., Jacchetti E., Soncini M., Raimondi M.T. Mechanosensing at the nuclear envelope by nuclear pore complex stretch activation and its effect in physiology and pathology. Front Physiol 2019; 10: 896, https://doi.org/10.3389/fphys.2019.00896.
- Elosegui-Artola A., Andreu I., Beedle A.E.M., Lezamiz A., Uroz M., Kosmalska A.J., Oria R., Kechagia J.Z., Rico-Lastres P., Le Roux A.L., Shanahan C.M., Trepat X., Navajas D., Garcia-Manyes S., Roca-Cusachs P. Force triggers YAP nuclear entry by regulating transport across nuclear pores. Cell 2017; 171(6): 1397–1410.e14, https://doi.org/10.1016/j.cell.2017.10.008.
- Knockenhauer K.E., Schwartz T.U. The nuclear pore complex as a flexible and dynamic gate. Cell 2016; 164(6): 1162–1171, https://doi.org/10.1016/j.cell.2016.01.034.
- Irianto J., Swift J., Martins R.P., McPhail G.D., Knight M.M., Discher D.E., Lee D.A. Osmotic challenge drives rapid and reversible chromatin condensation in chondrocytes. Biophys J 2013; 104: 759–769, https://doi.org/10.1016/j.bpj.2013.01.006.
- Heo S.J., Thorpe S.D., Driscoll T.P., Duncan R.L., Lee D.A., Mauck R.L. Biophysical regulation of chromatin architecture instills a mechanical memory in mesenchymal stem cells. Sci Rep 2015; 5: 16895, https://doi.org/10.1038/srep16895.
- Enyedi B., Jelcic M., Niethammer P. The cell nucleus serves as a mechanotransducer of tissue damage-induced inflammation. Cell 2016; 165(5): 1160–1170, https://doi.org/10.1016/j.cell.2016.04.016.
- Heo S.J., Cosgrove B.D., Dai E.N., Mauck R.L. Mechano-adaptation of the stem cell nucleus. Nucleus 2018; 9(1): 9–19, https://doi.org/10.1080/19491034.2017.1371398.
- Sen B., Xie Z., Case N., Thompson W.R., Uzer G., Styner M., Rubin J. mTORC2 regulates mechanically induced cytoskeletal reorganization and lineage selection in marrow-derived mesenchymal stem cells. J Bone Miner Res 2014; 29(1): 78–89, https://doi.org/10.1002/jbmr.2031.
- Cummings G.S., Tillman L.J. Remodeling of dense connective tissue in normal adult tissues. In: Dynamics of human biologic tissues contemporary perspectives in rehabilitation. Vol. 8. Philadelphia; 1992; p. 45–73.
- Sun X., Li F., Ma X., Ma J., Zhao B., Zhang Y., Li Y., Lv J., Meng X. The effects of combined treatment with naringin and treadmill exercise on osteoporosis in ovariectomized rats. Sci Rep 2015; 5: 13009, https://doi.org/10.1038/srep13009.
- Ju Y.I., Sone T., Ohnaru K., Tanaka K., Fukunaga M. Effect of swimming exercise on three-dimensional trabecular bone microarchitecture in ovariectomized rats. J Appl Physiol (1985) 2015; 119(9): 990–997, https://doi.org/10.1152/japplphysiol.00147.2015.
- Hanson A.D., Marvel S.W., Bernacki S.H., Banes A.J., van Aalst J., Loboa E.G. Osteogenic effects of rest inserted and continuous cyclic tensile strain on hASC lines with disparate osteodifferentiation capabilities. Ann Biomed Eng 2009; 37(5): 955–965, https://doi.org/10.1007/s10439-009-9648-7.
- Ageberg E., Engstrom G., Gerhardsson de Verdier M., Rollof J., Roos E.M., Lohmander L.S. Effect of leisure time physical activity on severe knee or hip osteoarthritis leading to total joint replacement: a population-based prospective cohort study. BMC Musculoskelet Disord 2012; 13: 73, https://doi.org/10.1186/1471-2474-13-73.
- Williams P.T. Effects of running and walking on osteoarthritis and hip replacement risk. Med Sci Sports Exerc 2013; 45(7): 1292–1297, https://doi.org/10.1249/MSS.0b013e3182885f26.
- Krauss I., Steinhilber B., Haupt G., Miller R., Martus P., Janssen P. Exercise therapy in hip osteoarthritis — a randomized controlled trial. Dtsch Arztebl Int 2015; 111(35–36): 592–599, https://doi.org/10.3238/arztebl.2014.0592.
- Quintrec J.L., Verlhac B., Cadet C., Breville P., Vetel J.M., Gauvain J.B., Jeandel C., Maheu E. Physical exercise and weight loss for hip and knee osteoarthritis in very old patients: a systematic review of the literature. Open Rheumatol J 2014; 8: 89–95, https://doi.org/10.2174/1874312901408010089.
- Svege I., Nordsletten L., Fernandes L., Risberg M.A. Exercise therapy may postpone total hip replacement surgery in patients with hip osteoarthritis: a long-term follow-up of a randomised trial. Ann Rheum Dis 2015; 74(1): 164–169, https://doi.org/10.1136/annrheumdis-2013-203628.
- Yourek G., McCormick S.M., Mao J.J., Reilly G.C. Shear stress induces osteogenic differentiation of human mesenchymal stem cells. Regen Med 2010; 5(5): 713–724, https://doi.org/10.2217/rme.10.60.
- Uzer G., Pongkitwitoon S., Ete Chan M., Judex S. Vibration induced osteogenic commitment of mesenchymal stem cells is enhanced by cytoskeletal remodeling but not fluid shear. J Biomech 2013; 46(13): 2296–2302, https://doi.org/10.1016/j.jbiomech.2013.06.008.
- Lee H.J., Choi B.H., Min B.H., Son Y.S., Park S.R. Low-intensity ultrasound stimulation enhances chondrogenic differentiation in alginate culture of mesenchymal stem cells. Artif Organs 2006; 30(9): 707–715, https://doi.org/10.1111/j.1525-1594.2006.00288.x.
- Weinheimer-Haus E.M., Judex S., Ennis W.J., Koh T.J. Low-intensity vibration improves angiogenesis and wound healing in diabetic mice. PLoS One 2014; 9(3): e91355, https://doi.org/10.1371/journal.pone.0091355.
- Uzer G., Thompson W.R., Sen B., Xie Z., Yen S.S., Miller S., Bas G., Styner M., Rubin C.T., Judex S., Burridge K., Rubin J. Cell mechanosensitivity to extremely low-magnitude signals is enabled by a LINCed nucleus. Stem Cells 2015; 33(6): 2063–2076, https://doi.org/10.1002/stem.2004.
- Francois M., Baldi J.C., Manning P.J., Lucas S.J., Hawley J.A., Williams M.J., Cotter J.D. ‘Exercise snacks’ before meals: a novel strategy to improve glycaemic control in individuals with insulin resistance. Diabetologia 2014; 57(7): 1437–1445, https://doi.org/10.1007/s00125-014-3244-6.
- Iwawaki Y., Mizusawa N., Iwata T., Higaki N., Goto T., Watanabe M., Tomotake Y., Ichikawa T., Yoshimoto K. Mir-494-3p induced by compressive force inhibits cell proliferation in MC3T3-E1 cells. J Biosci Bioeng 2015; 120(4): 456–462, https://doi.org/10.1016/j.jbiosc.2015.02.006.
- Wei F.L., Wang J.H., Ding G., Yang S.Y., Li Y., Hu Y.J., Wang S.L. Mechanical force-induced specific microRNA expression in human periodontal ligament stem cells. Cells Tissues Organs 2014; 199(5–6): 353–363, https://doi.org/10.1159/000369613.
- Chang M., Lin H., Luo M., Wang J., Han G. Integrated miRNA and mRNA expression profiling of tension force-induced bone formation in periodontal ligament cells. In Vitro Cell Dev Biol Anim 2015; 51(8): 797–807, https://doi.org/10.1007/s11626-015-9892-0.
- Mokhov D.E., Tregubova E.S., Kuzmina Yu.O., Potekhina Yu.P. Possibility of using osteopathic methods of treatment in infants of the first year of life. Voprosy prakticheskoj pediatrii 2018; 13(5): 91–97.
- Potekhina Yu.P., Mokhov D.E., Tregubova E.S. Etiology and pathogenesis of somatic dysfunctions. Klinicheskaya patofiziologiya 2017; 23(4): 16–26.
- Fryer G. Somatic dysfunction. International J Osteopath Med 2016; 22: 52–63, https://doi.org/10.1016/j.ijosm.2016.02.002.
- Tozzi P. A unifying neuro-fasciagenic model of somatic dysfunction: underlying mechanisms and treatment — part I. J Bodyw Mov Ther 2015; 19(2): 310–326, https://doi.org/10.1016/j.jbmt.2015.01.001.
- Tozzi P. A unifying neuro-fasciagenic model of somatic dysfunction — underlying mechanisms and treatment — part II. J Bodyw Mov Ther 2015; 19(3): 526–543, https://doi.org/10.1016/j.jbmt.2015.03.002.
- Liem T. A.T. Still’s osteopathic lesion theory and evidence-based models supporting the emerged concept of somatic dysfunction. J Am Osteopath Assoc 2016; 116(10): 654–661, https://doi.org/10.7556/jaoa.2016.129.
- Kramp M.E. Combined manual therapy techniques for the treatment of women with infertility: a case series. J Am Osteopath Assoc 2012; 112(10): 680–684.
- Chaudhry H., Schleip R., Ji Z., Bukiet B., Maney M., Findley T. Three-dimensional mathematical model for deformation of human fasciae in manual therapy. J Am Osteopath Assoc 2008; 108(8): 379–390, https://doi.org/10.7556/jaoa.2008.108.8.379.
- Tozzi P. Selected fascial aspects of osteopathic practice. J Bodyw Mov Ther 2012; 16(4): 503–519, https://doi.org/10.1016/j.jbmt.2012.02.003.
- McGlone F., Cerritelli F., Walker S., Esteves J. The role of gentle touch in perinatal osteopathic manual therapy. Neurosci Biobehav Rev 2017; 72: 1–9, https://doi.org/10.1016/j.neubiorev.2016.11.009.
- Bordoni B., Zanier E. Understanding fibroblasts in order to comprehend the osteopathic treatment of the fascia. Evid Based Complement Alternat Med 2015; 2015: 860934, https://doi.org/10.1155/2015/860934.
- Potekhina Yu.P. Role of connective tissue in the body. Rossijskij osteopaticheskij zhurnal 2015; 3–4: 92–104.
- Meltzer K.R., Standley P.R. Modeled repetitive motion strain and indirect osteopathic manipulative techniques in regulation of human fibroblast proliferation and interleukin secretion. J Am Osteopath Assoc 2007; 107(12): 527–536.
- Meltzer K.R., Cao T.V., Schad J.F., King H., Stoll S.T., Standley P.R. In vitro modeling of repetitive motion injury and myofascial release. J Bodyw Mov Ther 2010; 14(2): 162–171, https://doi.org/10.1016/j.jbmt.2010.01.002.
- Thompson W.R., Rubin C.T., Rubin J. Mechanical regulation of signaling pathways in bone. Gene 2012; 503(2): 179–193, https://doi.org/10.1016/j.gene.2012.04.076.
- Weaver V.M. Cell and tissue mechanics: the new cell biology frontier. Mol Biol Cell 2017; 28(14): 1815–1818, https://doi.org/10.1091/mbc.e17-05-0320.
- Hernández-Hernández V., Rueda D., Caballero L., Alvarez-Buylla E.R., Benítez M. Mechanical forces as information: an integrated approach to plant and animal development. Front Plant Sci 2014; 5: 265, https://doi.org/10.3389/fpls.2014.00265.
- Zhang Y., Yu J., Bomba H.N., Zhu Y., Gu Z. Mechanical force-triggered drug delivery. Chem Rev 2016; 116(19): 12536–12563, https://doi.org/10.1021/acs.chemrev.6b00369.
- Argentati C., Morena F., Tortorella I., Bazzucchi M., Porcellati S., Emiliani C., Martino S. Insight into mechanobiology: how stem cells feel mechanical forces and orchestrate biological functions. Int J Mol Sci 2019; 20(21): 5337, https://doi.org/10.3390/ijms20215337.
- Eckes B., Krieg T., Wickstroom S.A. Role of integrin signalling through integrin-linked kinase in skin physiology and pathology. Exp Dermatol 2014; 23(7): 453–456, https://doi.org/10.1111/exd.12429.